BBYCT-133 Solved Assignment
- a) Answer in one word:
i) The green plants which constitute the first trophic level of the food chain.
ii) A stage in which communities reach a stage of equilibrium.
iii) The organism that is eaten up by a predator.
b) Define the following:
i) Biome
ii) Net primary productivity
iii) Population
i) Biome
ii) Net primary productivity
iii) Population
c) Match the following:
i) Aquatic plants
a) Rank designating an organism
ii) Synecology
b) Binomial nomenclature
iii) Taxon
c) Hydrosere
iv) Linnaeus
d) Population/community
i) Aquatic plants
a) Rank designating an organism
ii) Synecology
b) Binomial nomenclature
iii) Taxon
c) Hydrosere
iv) Linnaeus
d) Population/community
- a) Describe the soil profile with the help of a well labelled diagram.
b) Enlist the major components of an ecosystem. Explain the importance of these components for the functioning of an ecosystem?
-
What is ecological succession? Explain the phenomenon giving an example of a desert community.
-
Describe the adaptations seen in xerophytes with the help of examples and well labelled diagrams.
-
a) Write notes on fidelity and importance value index.
b) What are the different functions of herbarium? -
a) Differentiate between alpha and omega taxonomy.
b) What are the salient features of structure of taxonomical hierarchy? -
Outline Bentham and Hooker’s system of classification. Mention the advantages and disadvantages of the system.
-
Discuss the different types of terrestrial and aquatic ecosystems.
-
a) Write a note on pyramid of energy.
b) Discuss the vegetation pattern of Western Himalayas? -
Write short notes on:
i) Importance of endemics
ii) Xerarch
iii) Systema Naturae
iv) Therophytes
Answer:
Question:-1(a)
Answer:
i) The green plants which constitute the first trophic level of the food chain. – Producers
ii) A stage in which communities reach a stage of equilibrium.- Climax
iii) The organism that is eaten up by a predator.- Prey
Question:-1(b)
Define the following: i) Biome ii) Net primary productivity iii) Population
Answer:
i) Biome:
A biome is a large-scale ecological community characterized by distinct climate, vegetation, and animal life, shaped by factors like temperature and precipitation. Examples include tropical rainforests, deserts, and tundras. Biomes encompass multiple ecosystems and represent global patterns of biodiversity, reflecting adaptations to specific environmental conditions.
A biome is a large-scale ecological community characterized by distinct climate, vegetation, and animal life, shaped by factors like temperature and precipitation. Examples include tropical rainforests, deserts, and tundras. Biomes encompass multiple ecosystems and represent global patterns of biodiversity, reflecting adaptations to specific environmental conditions.
ii) Net Primary Productivity (NPP):
Net primary productivity refers to the amount of energy (in the form of organic matter) that plants produce through photosynthesis, minus the energy they use for their own respiration. Measured typically in grams of carbon per square meter per year, NPP indicates the biomass available to herbivores and other consumers in an ecosystem, serving as a key metric of ecosystem productivity.
Net primary productivity refers to the amount of energy (in the form of organic matter) that plants produce through photosynthesis, minus the energy they use for their own respiration. Measured typically in grams of carbon per square meter per year, NPP indicates the biomass available to herbivores and other consumers in an ecosystem, serving as a key metric of ecosystem productivity.
iii) Population:
A population is a group of individuals of the same species living in a specific area at a given time, capable of interbreeding. It is a fundamental unit in ecology, studied for its size, density, and dynamics (e.g., birth and death rates). Populations interact with their environment and other species, influencing ecological processes like competition and predation.
A population is a group of individuals of the same species living in a specific area at a given time, capable of interbreeding. It is a fundamental unit in ecology, studied for its size, density, and dynamics (e.g., birth and death rates). Populations interact with their environment and other species, influencing ecological processes like competition and predation.
Question:-1(c)
Match the following: i) Aquatic plants a) Rank designating an organism ii) Synecology b) Binomial nomenclature iii) Taxon c) Hydrosere iv) Linnaeus d) Population/community
Answer:
- i) Aquatic plants – c) Hydrosere
- ii) Synecology – d) Population/community
- iii) Taxon – a) Rank designating an organism
- iv) Linnaeus – b) Binomial nomenclature
Question:-2(a)
Describe the soil profile with the help of a well labelled diagram.
Answer:
1. Introduction to Soil Profile
A soil profile is a vertical cross-section of soil from the surface down to the bedrock, revealing distinct layers or horizons that differ in physical, chemical, and biological properties. It represents the result of soil-forming processes—weathering, organic matter accumulation, and mineral translocation—over time. Typically studied in pedology, the soil profile provides insight into soil composition, fertility, and suitability for agriculture or ecosystem support. These horizons, labeled O, A, E, B, C, and R, reflect stages of soil development influenced by climate, organisms, topography, parent material, and time, known as the five soil-forming factors.
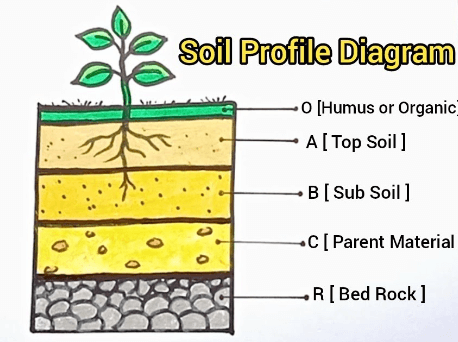
2. Horizons of the Soil Profile
The soil profile comprises several horizons, each with unique characteristics. The O horizon is the uppermost layer, rich in organic matter such as decomposing leaves, twigs, and microorganisms. It is dark, loose, and found in forested areas, though absent in heavily cultivated soils. Below it lies the A horizon, or topsoil, a mix of organic matter and minerals, typically dark brown due to humus. This fertile layer supports plant roots and is critical for agriculture.
The E horizon, present in some soils, is a zone of eluviation where minerals like iron and aluminum are leached downward by water, leaving a pale, sandy layer. The B horizon, or subsoil, accumulates these leached materials, appearing reddish or yellowish due to iron oxides or clay. It is denser and less fertile than the A horizon. The C horizon consists of weathered parent material—partially broken rock fragments—with minimal organic content. Finally, the R horizon is the unweathered bedrock, such as granite or limestone, marking the soil profile’s base.
3. Processes Shaping the Soil Profile
Soil horizons form through dynamic processes. Weathering breaks down parent material (C horizon) into smaller particles, initiating soil development. Physical weathering (e.g., freeze-thaw cycles) and chemical weathering (e.g., oxidation) contribute to this breakdown. Organic matter accumulation in the O and A horizons results from plant and animal decomposition, mediated by microbes, enhancing soil fertility. Leaching and translocation move minerals and nutrients downward, forming the E and B horizons. For instance, water percolating through the A horizon carries clay or iron to the B horizon, altering texture and color. These processes, influenced by environmental factors, create the stratified profile over centuries.
4. Diagram of the Soil Profile
A well-labeled diagram enhances understanding of the soil profile’s structure. Diagram Description: Imagine a vertical rectangular section divided into six horizontal layers. At the top, the O horizon is a thin, dark brown layer with leaf litter icons. Below, the A horizon is thicker, dark brown, with root symbols indicating topsoil. The E horizon, if present, is a light gray, sandy band. The B horizon follows, wider and reddish-brown, with clay particle icons showing accumulation. The C horizon is a thick, pale layer with rock fragments, transitioning to the R horizon, a solid gray bedrock base. Labels on the right identify each horizon (O, A, E, B, C, R), with arrows showing leaching from A to B. This visual depicts the gradual transition from organic-rich surface to mineral-dominated depths.
5. Significance of the Soil Profile
The soil profile is vital for ecological and human purposes. The O and A horizons support plant growth by providing nutrients and water retention, crucial for agriculture and forestry. The B horizon’s structure affects drainage and root penetration, while the C horizon influences soil depth and stability. Understanding the profile aids in land management—farmers assess topsoil quality, while engineers evaluate bedrock for construction. Ecologically, it sustains microbial life and nutrient cycling, linking aboveground ecosystems to underground processes. Variations in profiles (e.g., forest vs. desert soils) reflect local conditions, guiding conservation efforts.
Conclusion
In summary, the soil profile is a layered testament to soil formation, with horizons O, A, E, B, C, and R each playing distinct roles. From the organic-rich O horizon to the bedrock R horizon, these layers emerge through weathering, leaching, and organic activity, as depicted in the described diagram. This structure underpins soil’s ecological and practical importance, supporting life and human activities. By studying the soil profile, we gain a deeper appreciation of Earth’s dynamic surface, informing sustainable practices in agriculture, construction, and environmental stewardship.
Question:-2(b)
Enlist the major components of an ecosystem. Explain the importance of these components for the functioning of an ecosystem?
Answer:
1. Introduction to Ecosystem Components
An ecosystem is a dynamic system comprising living organisms and their non-living environment, interacting to maintain life processes. It functions through the interplay of its major components, broadly categorized into biotic (living) and abiotic (non-living) elements. These components—producers, consumers, decomposers, and abiotic factors like sunlight, water, and soil—are essential for energy flow, nutrient cycling, and ecological balance. Understanding their roles reveals how ecosystems sustain biodiversity and support life, from forests to oceans, emphasizing their interdependence and collective contribution to ecosystem stability.
2. Biotic Components: Producers
Producers, primarily green plants, algae, and cyanobacteria, form the foundation of ecosystems. They use photosynthesis to convert sunlight, carbon dioxide, and water into glucose, producing oxygen as a byproduct. This process harnesses solar energy, making it available to other organisms. In terrestrial ecosystems like grasslands, grasses are key producers, while in aquatic systems, phytoplankton dominate.
Importance: Producers are the entry point for energy into the ecosystem, constituting the first trophic level. They support herbivores and, indirectly, higher trophic levels, ensuring energy transfer. Their oxygen production sustains aerobic life, and their biomass contributes to habitat structure, such as trees in forests providing shelter. Without producers, ecosystems would collapse due to lack of energy and oxygen.
3. Biotic Components: Consumers
Consumers are organisms that cannot produce their own food and rely on others for energy. They include primary consumers (herbivores like rabbits), secondary consumers (carnivores like frogs), and tertiary consumers (top predators like eagles). Omnivores, such as humans, span multiple levels. Consumers are heterotrophs, acquiring energy by feeding on producers or other consumers.
Importance: Consumers regulate population dynamics and energy flow. Herbivores prevent producer overgrowth, maintaining vegetation balance, while predators control herbivore numbers, preventing overgrazing. This trophic interaction sustains biodiversity and ecosystem resilience. For example, wolves in Yellowstone National Park influence elk populations, indirectly benefiting plant communities, illustrating consumers’ cascading effects on ecosystem health.
4. Biotic Components: Decomposers
Decomposers, including bacteria, fungi, and detritivores like earthworms, break down dead organic matter and waste. They decompose plant litter, animal carcasses, and feces, converting complex organic compounds into simpler substances like carbon dioxide, water, and nutrients.
Importance: Decomposers are vital for nutrient cycling, recycling elements like nitrogen and phosphorus back into the soil or water for producer uptake. This prevents nutrient depletion and waste accumulation, maintaining soil fertility and ecosystem productivity. In a forest, fungi decomposing fallen logs enrich the soil, supporting new plant growth. Without decomposers, ecosystems would stagnate, choked by undecomposed matter.
5. Abiotic Components: Physical Environment
Abiotic components encompass non-living factors: sunlight, water, air, soil, temperature, and minerals. Sunlight drives photosynthesis, water is a medium for life processes, air provides oxygen and carbon dioxide, and soil offers nutrients and anchorage. Temperature and climate influence species distribution and metabolic rates.
Importance: Abiotic factors set the stage for biotic interactions. Sunlight powers producers, initiating energy flow, while water availability determines ecosystem type (e.g., desert vs. wetland). Soil composition affects plant growth, influencing food chains. In a coral reef, warm water and sunlight support coral photosynthesis, sustaining marine life. Abiotic stability ensures biotic components thrive, while disruptions (e.g., drought) can destabilize ecosystems.
6. Interdependence of Components
The ecosystem’s functioning hinges on the interplay of biotic and abiotic components. Producers rely on abiotic factors like sunlight and soil nutrients, consumers depend on producers for food, and decomposers recycle nutrients back to the abiotic environment. This cyclic relationship ensures energy transfer (via food chains) and matter recycling (via biogeochemical cycles). For instance, in a pond, algae (producers) use sunlight and water, fish (consumers) eat algae, and bacteria (decomposers) break down dead fish, returning nutrients to the water.
Importance: Interdependence maintains equilibrium. Disruption in one component—say, pollution killing decomposers—halts nutrient cycling, affecting producers and consumers, leading to ecosystem collapse. This synergy underscores the delicate balance sustaining life.
Conclusion
In essence, ecosystems thrive through the integration of producers, consumers, decomposers, and abiotic factors. Producers initiate energy flow, consumers regulate it, decomposers recycle nutrients, and abiotic elements provide the foundation. Each component’s importance lies in its contribution to energy transfer, nutrient cycling, and stability, as seen in their interdependent roles. From sustaining plant growth to preventing waste buildup, these elements collectively ensure ecosystems function as self-regulating units, supporting biodiversity and life across diverse habitats. Their harmonious interaction highlights the resilience and complexity of natural systems.
Question:-3
What is ecological succession? Explain the phenomenon giving an example of a desert community.
Answer:
1. Definition of Ecological Succession
Ecological succession is the process by which an ecological community undergoes a predictable series of changes in species composition and structure over time, following a disturbance or the creation of a new habitat. It reflects the dynamic nature of ecosystems, where pioneer species colonize barren or disrupted areas, gradually giving way to more complex communities until a stable climax community emerges. Succession is driven by interactions between organisms and their environment, including competition, facilitation, and environmental modification. It occurs in two forms: primary succession, starting on bare, lifeless substrates (e.g., volcanic rock), and secondary succession, occurring after disturbances (e.g., fires) in areas with pre-existing soil.
2. Mechanisms Driving Succession
Succession unfolds through distinct mechanisms. Initially, pioneer species—hardy organisms like lichens or grasses—colonize harsh environments, tolerating extreme conditions. These species alter the habitat by stabilizing soil, adding organic matter, or changing moisture levels, a process called facilitation. Over time, they create conditions favorable for less tolerant species, such as shrubs, which outcompete pioneers due to better resource use (e.g., light, nutrients). This competition shifts the community composition. Environmental factors like climate and soil development further shape the trajectory, leading to increased biodiversity and complexity. Eventually, a climax community—stable and self-sustaining—establishes, adapted to local conditions.
3. Stages of Succession
Succession progresses through recognizable stages. In primary succession, it begins with a barren substrate, followed by pioneer colonization (e.g., lichens breaking down rock). Next, early successional species (e.g., grasses) establish, enriching soil with organic matter. Mid-successional stages see shrubs or small trees dominating as soil deepens and nutrients accumulate. Finally, the climax stage features a mature community (e.g., forests in temperate regions) with high species diversity and stability. In secondary succession, stages are similar but faster, as soil and seed banks persist post-disturbance. Each stage builds on the previous, reflecting gradual ecological transformation.
4. Example: Succession in a Desert Community
Desert succession illustrates primary succession in an arid environment. Consider a newly exposed sandy expanse after glacial retreat or tectonic activity. Initially, the substrate is bare, with extreme heat, low water, and no organic matter. Pioneer species like lichens or cyanobacteria colonize, forming crusts that stabilize sand and retain scant moisture. Their decomposition adds organic content, enabling hardy grasses (e.g., Aristida) to take root, their shallow roots binding soil further. Over decades, shrubs like creosote bush (Larrea tridentata) emerge, thriving in dry conditions and shading the ground, reducing evaporation. Their litter enhances soil fertility, supporting small perennials or cacti (e.g., Opuntia). After centuries, a climax desert community forms, featuring drought-adapted shrubs, cacti, and sparse trees like Joshua trees (Yucca brevifolia), balanced with the arid climate. Small mammals (e.g., kangaroo rats) and reptiles join, relying on plant cover and seeds.
Key Features: This succession is slow due to limited water and nutrients. Soil development is minimal, with shallow, sandy layers, and the climax community remains simple compared to wetter regions, reflecting desert constraints. Facilitation by pioneers and competition for scarce resources drive the process, culminating in a stable, resilient ecosystem.
5. Ecological Significance of Succession
Succession is vital for ecosystem recovery and development. It restores biodiversity after disturbances, as seen in deserts reclaiming barren land. It enhances soil formation, critical in deserts where pioneers initiate nutrient cycles. Succession also reflects resilience, allowing communities to adapt to changing conditions, such as climate shifts. In deserts, it supports specialized species, maintaining ecological roles like seed dispersal by animals. Understanding succession aids conservation, predicting how ecosystems recover from human impacts (e.g., mining) or natural events, ensuring sustainable management of fragile habitats.
Conclusion
Ecological succession is a transformative process where ecosystems evolve from barren states to stable communities through pioneer colonization, species replacement, and environmental modification. In a desert, this unfolds from cyanobacteria and grasses to a climax of shrubs and cacti, driven by facilitation and competition under harsh conditions. This phenomenon underscores nature’s ability to heal and adapt, building biodiversity and stability over time. The desert example highlights succession’s role in creating life-sustaining ecosystems in extreme environments, offering lessons in resilience and the intricate interplay of life and landscape.
Question:-4
Describe the adaptations seen in xerophytes with the help of examples and well labelled diagrams.
Answer:
1. Introduction to Xerophytes
Xerophytes are plants specifically adapted to thrive in arid environments where water availability is scarce, such as deserts, rocky terrains, or high-altitude dry zones. These conditions include low rainfall (often less than 250 mm annually), high evaporation rates, intense sunlight, and poor soil water retention. Xerophytes have evolved a range of structural, physiological, and behavioral adaptations to minimize water loss, maximize water uptake, and endure prolonged droughts. These adaptations ensure their survival and reproduction in habitats where most plants would perish, making them critical components of xeric ecosystems like the Sahara Desert or the Western Himalayas’ drier slopes.
2. Morphological Adaptations
Morphological adaptations refer to physical changes in xerophyte structure that enhance water conservation and drought resistance. Leaves are often reduced to spines or scales, as seen in cacti (e.g., Opuntia), minimizing surface area for transpiration while deterring herbivores. In plants like succulents (e.g., Aloe vera), thick, fleshy leaves or stems store water in specialized parenchyma cells, acting as reservoirs during dry periods. The epidermis is typically covered with a thick cuticle—a waxy layer—that reduces evaporation, as observed in Euphorbia species. Additionally, xerophytes like the creosote bush (Larrea tridentata) have small, leathery leaves with sunken stomata, concentrated on the leaf underside to limit water loss while still allowing gas exchange. Root systems are another key feature: shallow, widespread roots (e.g., in desert grasses) maximize absorption of brief rainfall, while deep taproots (e.g., in mesquite, Prosopis) access groundwater far below the surface.
3. Physiological Adaptations
Physiological adaptations involve internal mechanisms that regulate water use and metabolic processes. Xerophytes often employ Crassulacean Acid Metabolism (CAM) photosynthesis, a water-saving strategy seen in plants like agaves and cacti. In CAM, stomata open at night to take in CO₂ (when temperatures are cooler and humidity higher), storing it as malic acid, then close during the day to prevent transpiration while using the stored CO₂ for photosynthesis. Another adaptation is the ability to tolerate high cellular dehydration, as seen in resurrection plants (e.g., Selaginella lepidophylla), which curl up when dry and revive upon rehydration. Xerophytes also reduce metabolic rates during drought, entering dormancy to conserve energy, and many produce high concentrations of solutes (e.g., proline) to lower water potential, aiding water retention in salty or dry soils. These mechanisms ensure survival even when external water sources vanish for months.
4. Behavioral and Reproductive Adaptations
Behavioral adaptations in xerophytes include strategies to optimize water use and reproduction under unpredictable conditions. Many xerophytes, like desert annuals (e.g., California poppy, Eschscholzia californica), exhibit rapid life cycles, germinating, growing, and seeding within weeks of rare rainfall—an adaptation classifying them as therophytes. Seeds often have hard coats with dormancy mechanisms, remaining viable in soil for years until moisture triggers germination, ensuring reproduction aligns with favorable conditions. Some species, like the Joshua tree (Yucca brevifolia), shed leaves or branches during extreme drought to reduce water demand, a form of self-pruning. Orientation of leaves to minimize sun exposure, as in certain agaves, also reduces heat stress and evaporation. These adaptive behaviors enhance survival and propagation in erratic climates.
5. Ecological Role and Examples
Xerophytes play a vital ecological role in stabilizing arid ecosystems and supporting biodiversity. Their root systems prevent soil erosion in windy deserts, while their presence provides food and shelter for specialized fauna, such as the cactus wren nesting in saguaro cacti. Examples of xerophytes showcase their diversity: the barrel cactus (Ferocactus) stores water in its swollen stem, the saltbush (Atriplex) tolerates saline soils with salt-excreting glands, and the date palm (Phoenix dactylifera) combines deep roots with waxy fronds to thrive in oases. In the Western Himalayas, species like Caragana shrubs adapt to cold, dry high altitudes with small leaves and extensive roots. These plants not only survive but also shape their harsh environments, contributing to nutrient cycling and habitat formation.
Conclusion
Xerophytes exemplify nature’s resilience, adapting to water-scarce environments through an intricate blend of morphological, physiological, and behavioral traits. Their reduced leaves, water-storing tissues, and efficient photosynthetic pathways minimize loss and maximize uptake, while rapid life cycles and dormant seeds ensure reproductive success. These adaptations not only enable xerophytes to endure extreme conditions but also underscore their ecological importance in stabilizing arid landscapes and supporting life. From the spiny cacti of scorching deserts to the hardy shrubs of high-altitude dry zones, xerophytes demonstrate evolutionary ingenuity, offering insights into plant survival strategies and potential applications in agriculture or conservation in an increasingly arid world.
Question:-5(a)
Write notes on fidelity and importance value index.
Answer:
1. Introduction to Ecological Metrics
In ecology, understanding plant community structure and species interactions relies on quantitative tools like fidelity and the importance value index (IVI). These metrics assess species roles within ecosystems, aiding in biodiversity studies, conservation, and vegetation analysis. Fidelity measures a species’ loyalty to specific habitats, reflecting ecological specialization, while IVI quantifies a species’ overall dominance or significance in a community. Both are widely used in phytosociology and forest ecology to interpret plant distribution, abundance, and environmental relationships, offering insights into ecosystem dynamics and health.
2. Fidelity: Concept and Definition
Fidelity refers to the degree to which a plant species is consistently associated with a particular habitat or community type. It indicates how "faithful" a species is to specific ecological conditions, such as soil type, moisture, or climate. High fidelity means a species occurs almost exclusively in one community, while low fidelity suggests it appears across diverse habitats. Ecologists classify fidelity into categories: exclusive (restricted to one community), selective (prefers one but occurs elsewhere), preferential (more frequent in one), and indifferent (no preference).
Application and Measurement: Fidelity is assessed by comparing a species’ presence in a target community versus others, often using presence-absence data from quadrats. For example, Sphagnum moss has high fidelity to bogs due to its dependence on acidic, wet conditions. It’s calculated qualitatively or with indices like the phi coefficient, reflecting ecological specialization. This helps identify indicator species—plants signaling specific environmental conditions—crucial for habitat classification and monitoring changes.
3. Importance Value Index: Concept and Definition
The Importance Value Index (IVI) is a composite metric that quantifies a species’ ecological significance in a community, combining its abundance, frequency, and dominance. It provides a holistic view of a species’ role, often applied in forest or vegetation surveys to rank species’ contributions. IVI integrates three parameters: relative density (number of individuals relative to total), relative frequency (proportion of sample plots where the species occurs), and relative dominance (typically basal area or cover relative to total), each standardized to a percentage.
Calculation: IVI is the sum of these relative values, with a maximum of 300 (100 per component). For instance, in a forest, a tree like oak (Quercus robur) might have high IVI due to numerous individuals (density), widespread occurrence (frequency), and large canopy cover (dominance). This index highlights dominant species shaping community structure and resource use.
4. Significance and Uses of Fidelity
Fidelity is significant for understanding species-habitat relationships and ecological stability. Indicator Role: High-fidelity species, like Vaccinium uliginosum in tundra, signal specific conditions (e.g., cold, acidic soils), aiding in ecosystem classification. Conservation: Identifying faithful species helps prioritize habitats for protection, as their presence reflects environmental integrity. Succession Studies: Fidelity shifts during succession—pioneer species may have low fidelity, while climax species show higher fidelity—revealing community development stages. In practice, ecologists use fidelity to map vegetation zones, assess disturbance impacts, and predict responses to climate change, enhancing ecological management.
5. Significance and Uses of Importance Value Index
IVI’s importance lies in its ability to rank species’ ecological influence, guiding research and management. Community Analysis: In a savanna, a grass like Andropogon gerardii with high IVI indicates dominance, shaping grazing patterns and fire regimes. Resource Management: Foresters use IVI to identify key timber or keystone species, balancing exploitation and conservation. Biodiversity Assessment: Low IVI across many species suggests high diversity, while high IVI for few indicates monoculture risks. IVI’s quantitative nature makes it ideal for comparing sites, tracking succession, or evaluating restoration success, providing a snapshot of ecosystem structure.
Conclusion
Fidelity and the Importance Value Index are indispensable tools in ecological science, illuminating species’ roles within communities. Fidelity highlights habitat-specific associations, identifying indicator species and aiding conservation, as seen with bog-loving Sphagnum. IVI, blending density, frequency, and dominance, quantifies a species’ ecological weight, as with dominant oaks in forests. Together, they offer complementary perspectives—fidelity on specificity, IVI on prominence—enriching our understanding of vegetation patterns, ecosystem function, and environmental change. Their application in research and management underscores their value in sustaining biodiversity and ecological balance.
Question:-5(b)
What are the different functions of herbarium?
Answer:
Functions of a Herbarium
A herbarium is a collection of preserved plant specimens, systematically arranged, labeled, and stored for scientific study. Beyond mere storage, herbaria serve multiple critical functions in botanical research, education, conservation, and taxonomy. Here are the key functions:
-
Preservation of Plant Specimens
Herbaria act as repositories where plant specimens are dried, pressed, and mounted on sheets, preserving them for decades or centuries. This ensures a permanent record of plant diversity, including rare or extinct species, with details like collection date, location, and habitat noted on labels. For example, specimens of Silene stenophylla from a Siberian herbarium, over 30,000 years old, have been revived, showcasing preservation’s long-term value. -
Taxonomic Research and Identification
Herbaria are essential for taxonomy—the science of classifying plants. Researchers compare new specimens against preserved ones to identify species, describe new taxa, or revise classifications. Type specimens (original samples used to name species) are housed in herbaria, serving as reference standards. For instance, the Kew Herbarium aids in identifying tropical plants, supporting global taxonomic studies. -
Documentation of Biodiversity
By cataloging plants from diverse regions, herbaria document biodiversity and geographic distribution. They provide historical baselines, showing how flora has changed over time due to climate shifts or human activity. The Harvard University Herbaria, with millions of specimens, tracks North American plant ranges, revealing shifts like those caused by deforestation. -
Educational Resource
Herbaria serve as teaching tools for students, botanists, and educators. They offer hands-on learning about plant morphology, diversity, and identification. Universities like the University of Delhi use herbarium collections in botany courses, allowing students to study real specimens rather than relying solely on illustrations or digital images. -
Support for Conservation Efforts
Herbaria provide data for conserving endangered species and restoring habitats. Specimen records reveal past distributions, helping identify areas for reintroduction. For example, herbarium data on IUCN Red List plants guide efforts to protect species like the Hawaiian Hibiscus clayi, informing strategies against habitat loss or invasive species. -
Research on Plant Evolution and Ecology
Herbaria enable studies of evolutionary relationships and ecological adaptations by preserving specimens across time and space. DNA extraction from old specimens, as done with Arabidopsis samples, reveals genetic changes, while habitat notes on labels inform ecological studies, such as how desert plants adapted to aridity over millennia. -
Source of Ethnobotanical and Medicinal Knowledge
Many herbarium specimens include notes on traditional uses, supporting ethnobotanical research. Plants like Cinchona (source of quinine) in herbaria have historical records of medicinal use, guiding modern pharmacology. This function bridges cultural heritage and scientific discovery, preserving indigenous knowledge. -
Monitoring Environmental Change
Herbaria track environmental shifts, such as climate change or pollution effects, by comparing historical and current specimens. Phenological data (e.g., flowering times) from herbaria, like those at the Missouri Botanical Garden, show how warming climates alter plant cycles, aiding predictive models for ecosystem impacts.
Summary
The functions of a herbarium—preservation, taxonomic research, biodiversity documentation, education, conservation support, evolutionary studies, ethnobotanical insights, and environmental monitoring—make it a cornerstone of botanical science. Herbaria like those at Kew, Harvard, or local institutions are not static collections but dynamic resources driving research, education, and conservation, ensuring plant knowledge endures for future generations.
Question:-6(a)
Differentiate between alpha and omega taxonomy.
Answer:
Differentiation Between Alpha Taxonomy and Omega Taxonomy
Taxonomy, the science of classifying organisms, evolves through distinct phases, with alpha taxonomy and omega taxonomy representing different stages and approaches in this process. Here’s how they differ:
1. Definition and Scope
- Alpha Taxonomy: Alpha taxonomy is the initial stage of taxonomic study, focusing on the identification, naming, and basic classification of species based primarily on morphological characteristics. It aims to catalog and describe species diversity within a group, establishing a foundational inventory. For example, identifying a new plant species like Rosa indica based on flower shape and leaf structure is alpha taxonomy.
- Omega Taxonomy: Omega taxonomy is an advanced, integrative stage that builds on alpha taxonomy by incorporating detailed data from multiple disciplines—morphology, genetics, ecology, and physiology—to refine classifications and understand evolutionary relationships. It seeks a comprehensive synthesis, often revising earlier classifications, as seen in reclassifying species within the genus Quercus (oaks) using DNA evidence.
2. Objective
- Alpha Taxonomy: The primary goal is to discover, describe, and name new species, creating a preliminary framework. It answers "What species exist?" and assigns binomial names per Linnaean rules, emphasizing species delineation. For instance, Linnaeus’ work on Systema Naturae exemplifies alpha taxonomy by naming countless plants and animals.
- Omega Taxonomy: The objective is to achieve a holistic understanding of species, resolving phylogenetic relationships and taxonomic ambiguities. It asks "How are species related?" and aims for a stable, evolutionarily accurate classification, integrating diverse data to confirm or revise species status, like distinguishing cryptic species in Selaginella.
3. Methodology
- Alpha Taxonomy: Relies heavily on observable morphological traits—size, shape, color, and structure—examined via specimens (e.g., herbarium sheets) or field observations. Techniques are simpler, involving microscopes or hand lenses, with limited use of molecular tools. Describing Euphorbia milii based on its spiny stems and red bracts is a typical alpha approach.
- Omega Taxonomy: Employs advanced methods, combining morphology with molecular biology (DNA sequencing), biochemistry (protein analysis), and ecological data. It uses cladistics and phylogenetic trees to map evolutionary lineages. For example, omega taxonomy might use genetic markers to differentiate Pinus sylvestris subspecies, beyond mere needle length.
4. Data Utilization
- Alpha Taxonomy: Focuses on external, macroscopic features, often ignoring internal or genetic traits. It uses qualitative descriptions and illustrations, producing monographs or floras, like Bentham and Hooker’s classification of flowering plants based on floral morphology.
- Omega Taxonomy: Integrates extensive datasets—genomic sequences, karyotypes, reproductive behavior, and habitat preferences—offering a multidimensional view. It leverages bioinformatics and statistical tools, as seen in revising orchid genera using chloroplast DNA alongside flower shape.
5. Stage in Taxonomy
- Alpha Taxonomy: Represents the starting point, prevalent in unexplored regions or groups with unknown diversity (e.g., tropical rainforests). It’s ongoing where species remain undescribed, such as in deep-sea algae studies.
- Omega Taxonomy: Marks an endpoint or ideal, achieved after extensive alpha work, refining classifications with modern science. It’s prominent in well-studied groups like mammals or temperate flora, aiming for taxonomic stability, though rarely fully realized due to ongoing discoveries.
6. Examples and Application
- Alpha Taxonomy: Naming Lycopodium clavatum based on its club-shaped sporangia is alpha taxonomy, foundational for herbarium records and field guides.
- Omega Taxonomy: Reclassifying Arabidopsis species using genomic data to clarify evolutionary divergence exemplifies omega taxonomy, enhancing phylogenetic studies and conservation priorities.
7. Limitations
- Alpha Taxonomy: Limited by its reliance on morphology, it may overlook cryptic species (morphologically similar but genetically distinct) and lacks depth in evolutionary context.
- Omega Taxonomy: Requires sophisticated technology and expertise, making it resource-intensive and less accessible in underfunded regions, though it overcomes alpha’s superficiality.
Summary
Alpha taxonomy lays the groundwork by identifying and naming species through morphology, as seen in early botanical surveys, while omega taxonomy refines this through integrative, evolutionary analysis, leveraging modern tools like DNA sequencing. Alpha is the first step—broad, descriptive, and essential—whereas omega is the aspirational pinnacle, synthesizing diverse data for precision. Together, they represent taxonomy’s progression from discovery to understanding, each vital in its context.
Question:-6(b)
What are the salient features of structure of taxonomical hierarchy?
Answer:
Salient Features of the Structure of Taxonomic Hierarchy
Taxonomic hierarchy is a systematic framework used in biology to classify and organize living organisms into a series of nested ranks based on shared characteristics and evolutionary relationships. Developed by Carl Linnaeus and refined over time, it provides a structured approach to understanding biodiversity. Here are its key features:
1. Hierarchical Arrangement
The taxonomic hierarchy is organized into a series of ranks or levels, arranged from the broadest to the most specific. This nested structure ensures that each level encompasses the one below it, reflecting increasing specificity. The primary ranks, in descending order, are: Domain, Kingdom, Phylum, Class, Order, Family, Genus, and Species. For example, humans are classified as Domain: Eukarya, Kingdom: Animalia, Phylum: Chordata, down to Species: Homo sapiens. This hierarchy allows for a logical, step-wise classification of organisms.
2. Binomial Nomenclature
At the species level, the hierarchy employs binomial nomenclature, a two-part naming system combining the genus and species names (e.g., Panthera leo for lion). Introduced by Linnaeus, this feature ensures universal, unique identification of species across languages and regions, with the genus capitalized and species lowercase, both italicized. It anchors the hierarchy’s base, providing precision and consistency in scientific communication.
3. Progressive Specificity
Each rank in the hierarchy represents a progressively narrower group of organisms sharing more specific traits. For instance, Kingdom Animalia includes all animals, while Phylum Chordata narrows to those with a notochord, and Genus Felis specifies cats. This feature reflects evolutionary divergence, with higher ranks (e.g., Phylum) grouping organisms by broad characteristics (e.g., body plan) and lower ranks (e.g., Species) by fine details (e.g., reproductive compatibility), facilitating detailed classification.
4. Flexibility with Intermediate Ranks
The hierarchy is flexible, allowing intermediate ranks like Subphylum, Subclass, Infraorder, or Subfamily to accommodate complex diversity. For example, Subclass Eutheria (placental mammals) refines Class Mammalia, and Tribe Bambuseae fits between Family Poaceae and Genus Bambusa. This adaptability ensures the system can handle taxonomic complexity, such as in plants with vast morphological variation, enhancing resolution without disrupting the core structure.
5. Basis on Shared Characteristics
Classification within the hierarchy relies on shared morphological, anatomical, genetic, and ecological traits. Higher ranks group organisms by fundamental features (e.g., Kingdom Plantae for photosynthetic organisms), while lower ranks use finer distinctions (e.g., Family Cactaceae for succulent stems). Modern taxonomy increasingly incorporates phylogenetic data (evolutionary relationships), aligning the hierarchy with cladistics, as seen in reclassifying birds within Class Aves based on DNA evidence.
6. Universality and Standardization
The taxonomic hierarchy is universally accepted, providing a standardized framework across scientific communities globally. Governed by codes like the International Code of Nomenclature for algae, fungi, and plants (ICN) and the International Code of Zoological Nomenclature (ICZN), it ensures consistency in naming and ranking. For instance, Canis lupus (wolf) is recognized worldwide, preventing confusion from local names and fostering collaborative research.
7. Evolutionary Reflection
The hierarchy mirrors evolutionary history, grouping organisms by common ancestry. Domains (e.g., Bacteria, Archaea, Eukarya) reflect the deepest evolutionary splits, while genera and species denote recent divergences. This phylogenetic basis, enhanced by molecular data, allows the hierarchy to evolve—e.g., reclassifying fungi from Kingdom Plantae to Kingdom Fungi—ensuring it remains a dynamic tool aligned with scientific advancements.
8. Categorical Inclusivity
Each rank is inclusive, containing all subordinate taxa. A Family (e.g., Rosaceae) includes multiple genera (Rosa, Prunus), which in turn include species (Rosa indica, Prunus persica). This feature organizes biodiversity comprehensively, allowing ecologists, taxonomists, and conservationists to study relationships at any level, from broad ecological roles (e.g., Order Carnivora) to specific genetic studies (e.g., Species Panthera tigris).
Summary
The taxonomic hierarchy’s salient features—hierarchical arrangement, binomial nomenclature, progressive specificity, flexibility, shared characteristics, universality, evolutionary reflection, and inclusivity—create a robust, adaptable system for classifying life. From Domain to Species, it balances broad categorization with precise identification, as seen in examples like Homo sapiens or Felis catus. This structure not only organizes biodiversity but also supports research, conservation, and education by providing a universal, evolution-based framework.
Question:-7
Outline Bentham and Hooker’s system of classification. Mention the advantages and disadvantages of the system.
Answer:
Outline of Bentham and Hooker’s System of Classification
Bentham and Hooker’s system of classification is a natural system developed by British botanists George Bentham and Joseph Dalton Hooker in their monumental work, Genera Plantarum (1862–1883). Designed to classify flowering plants (angiosperms), it organizes them based on observable morphological characteristics, reflecting natural relationships. Below is an outline of its structure and key features:
1. Basis of Classification
The system relies on external morphological traits, primarily floral characters (e.g., number, arrangement, and fusion of sepals, petals, stamens, and carpels), alongside vegetative features (e.g., leaf arrangement). It aims to group plants with similar natural affinities, emphasizing shared physical attributes over evolutionary lineage, which was less understood at the time.
2. Major Divisions
Bentham and Hooker divided seed plants (Phanerogams or Spermatophytes) into three main classes:
- Dicotyledonae (Dicots): Plants with two cotyledons, net-veined leaves, and floral parts typically in fours or fives. Example: Rosa (rose).
- Gymnospermae: Naked-seed plants with exposed ovules, placed between dicots and monocots. Example: Pinus (pine).
- Monocotyledonae (Monocots): Plants with one cotyledon, parallel-veined leaves, and floral parts usually in threes. Example: Oryza (rice).
3. Subdivision of Dicotyledonae
Dicots, the largest group, are further divided into three subclasses based on floral structure:
- Polypetalae: Petals free and separate, with a distinct calyx and corolla. Example: Ranunculus (buttercup). Subdivided into series like Thalamiflorae, Disciflorae, and Calyciflorae based on ovary position and floral disc.
- Gamopetalae: Petals fused into a tube or united, often with advanced floral symmetry. Example: Solanum (potato). Divided into series like Inferae, Heteromerae, and Bicarpellatae based on ovary and carpel features.
- Monochlamydeae (Apetalae): Flowers lacking petals or with a single perianth whorl. Example: Quercus (oak). Includes simpler, often wind-pollinated plants.
4. Hierarchical Structure
The system uses a hierarchy of Classes, Subclasses, Series, Orders (Families), Genera, and Species. For instance, Dicotyledonae → Polypetalae → Thalamiflorae → Ranunculaceae → Ranunculus. They recognized 202 orders (equivalent to modern families), detailing 7,569 genera and numerous species, based on specimen analysis from Kew Gardens and other herbaria.
5. Treatment of Gymnosperms and Monocots
- Gymnosperms: Treated as a distinct class between dicots and monocots, reflecting their naked seeds and woody nature, though not extensively subdivided (e.g., families like Pinaceae).
- Monocots: Organized into series like Petaloideae (petal-like perianth) and Glumaceae (reduced flowers, e.g., grasses), emphasizing perianth and inflorescence variations.
6. Natural System Approach
Unlike artificial systems (e.g., Linnaeus’ sexual system focusing solely on stamens and pistils), Bentham and Hooker aimed for a natural classification, grouping plants by overall similarity in multiple traits, suggesting evolutionary affinity, though without explicit phylogenetic intent due to pre-Darwinian origins.
Advantages of Bentham and Hooker’s System
- Comprehensive and Practical: It cataloged a vast number of angiosperms (over 7,500 genera), serving as a practical reference for botanists, especially in herbaria like Kew, where specimens were physically organized by this system.
- Natural Relationships: By using multiple morphological traits, it reflected natural affinities better than artificial systems, grouping related plants (e.g., Rosaceae) based on shared features, aiding identification.
- Detailed Descriptions: The Genera Plantarum provided meticulous genus-level descriptions, making it a foundational taxonomic resource still consulted for historical context.
- Ease of Use: Its reliance on observable characters (e.g., petal fusion) made it accessible to botanists without advanced tools, facilitating fieldwork and education.
Disadvantages of Bentham and Hooker’s System
- Lack of Evolutionary Basis: Developed before Darwin’s theory gained wide acceptance, it doesn’t reflect phylogenetic relationships, placing gymnosperms oddly between dicots and monocots, unlike modern cladistic systems.
- Overemphasis on Morphology: Reliance on external traits ignored internal anatomy, physiology, or genetics, missing cryptic species or evolutionary links (e.g., Monochlamydeae’s heterogeneity).
- Static Framework: It struggles to incorporate new species or molecular data, rendering it outdated compared to phylogenetic systems like APG (Angiosperm Phylogeny Group).
- Complexity and Subjectivity: Subdivisions (e.g., series within Polypetalae) can be intricate and subjective, depending on interpretation of floral variations, complicating consistent application.
Summary
Bentham and Hooker’s system, a landmark natural classification, organizes flowering plants into Dicotyledonae, Gymnospermae, and Monocotyledonae, with detailed subdivisions based on morphology. Its strengths—comprehensiveness and practicality—made it invaluable historically, while its weaknesses—lack of evolutionary grounding and rigidity—limit its modern use. It remains a testament to 19th-century botany, bridging artificial and phylogenetic approaches.
Question:-8
Discuss the different types of terrestrial and aquatic ecosystems.
Answer:
Ecosystems are dynamic communities of living organisms interacting with each other and their physical environment. They can be broadly categorized into terrestrial ecosystems (land-based) and aquatic ecosystems (water-based).
Terrestrial Ecosystems
Terrestrial ecosystems are defined by climate, soil type, vegetation, and geographical location. Here are the primary types:
-
Forests
- Description: Dominated by trees and dense vegetation, forests vary based on climate.
- Types:
- Tropical Rainforests: Found near the equator (e.g., Amazon), with high rainfall, warm temperatures, and exceptional biodiversity (e.g., monkeys, jaguars, orchids).
- Temperate Forests: Occur in regions with distinct seasons (e.g., North America, Europe), featuring deciduous trees (e.g., oak, maple) or evergreens, and animals like deer and bears.
- Boreal Forests (Taiga): Found in colder northern regions (e.g., Canada, Siberia), dominated by coniferous trees (e.g., pine, spruce) and adapted to long winters (e.g., moose, wolves).
- Key Features: High biomass, carbon storage, and layered structure (canopy, understory, forest floor).
-
Grasslands
- Description: Vast areas dominated by grasses rather than large trees, due to moderate rainfall insufficient for forest growth.
- Types:
- Savannas: Tropical or subtropical with scattered trees (e.g., African savanna), home to grazers like zebras and predators like lions.
- Temperate Grasslands: Found in regions like the North American prairies or Russian steppes, with fertile soils supporting grasses and animals like bison or prairie dogs.
- Key Features: Fire and grazing maintain grass dominance; highly productive for agriculture.
-
Deserts
- Description: Arid regions with low precipitation (<250 mm/year), extreme temperatures, and sparse vegetation.
- Types:
- Hot Deserts: Like the Sahara, with scorching days and cold nights, hosting adapted species (e.g., cacti, camels, scorpions).
- Cold Deserts: Like the Gobi, with frigid winters and drought-tolerant shrubs.
- Key Features: Water scarcity drives adaptations like water storage (succulents) or nocturnal behavior.
-
Tundra
- Description: Cold, treeless regions with short growing seasons and permafrost (frozen soil).
- Types:
- Arctic Tundra: Near the poles (e.g., Alaska, Siberia), with mosses, lichens, and animals like polar bears or reindeer.
- Alpine Tundra: Found at high altitudes (e.g., Himalayas), with similar vegetation but no permafrost.
- Key Features: Low biodiversity, harsh winds, and adaptations to cold (e.g., thick fur, small stature).
-
Mountains
- Description: Ecosystems varying with altitude, from forested bases to tundra-like peaks.
- Key Features: Diverse microclimates, species like mountain goats or snow leopards, and steep gradients affecting soil and vegetation.
Aquatic Ecosystems
Aquatic ecosystems are classified by water type (freshwater or saltwater), depth, and flow. They cover about 71% of Earth’s surface and include:
-
Freshwater Ecosystems
- Description: Low salt content (<1%), critical for drinking water and supporting diverse life.
- Types:
- Rivers and Streams: Flowing water systems (e.g., Mississippi River), with organisms like fish (salmon), insects, and algae adapted to currents.
- Lakes and Ponds: Standing water bodies (e.g., Lake Baikal), varying in size and depth, hosting plankton, fish (e.g., trout), and amphibians.
- Wetlands: Saturated areas (e.g., swamps, marshes, bogs) with standing water or soggy soils, rich in biodiversity (e.g., herons, cattails, alligators).
- Key Features: Nutrient cycling, seasonal changes, and sensitivity to pollution.
-
Marine Ecosystems
- Description: Saltwater environments (salinity ~3.5%), covering oceans and seas, with immense biodiversity.
- Types:
- Coral Reefs: Biodiverse “rainforests of the sea” (e.g., Great Barrier Reef), built by calcium carbonate from corals, supporting fish, mollusks, and sharks.
- Open Ocean (Pelagic Zone): Vast, deep waters (e.g., Pacific Ocean), with zones like the sunlit epipelagic (e.g., tuna, dolphins) and dark abyssal (e.g., anglerfish).
- Coastal Zones: Near shorelines (e.g., estuaries, mangroves), mixing freshwater and saltwater, nurturing crabs, oysters, and migratory birds.
- Deep Sea: Below 1,000 meters, with extreme pressure and no light, inhabited by bioluminescent species (e.g., squid, tube worms near hydrothermal vents).
- Key Features: High productivity in coastal areas, vast unexplored regions, and complex food webs.
-
Transitional Ecosystems (Brackish Water)
- Description: Areas where freshwater and saltwater mix, like estuaries or deltas.
- Key Features: Fluctuating salinity, supporting unique species (e.g., mangroves, crabs, and juvenile fish using them as nurseries).
Key Differences and Interactions
- Terrestrial vs. Aquatic: Terrestrial ecosystems rely heavily on soil and climate, while aquatic ones depend on water chemistry (salinity, pH, oxygen levels) and depth/light penetration.
- Energy Flow: Both feature producers (plants, algae), consumers (herbivores, carnivores), and decomposers, but aquatic systems often depend on phytoplankton as a base, while terrestrial systems rely on larger plants.
- Interconnectedness: Many species (e.g., amphibians, migratory birds) link terrestrial and aquatic ecosystems, and processes like runoff tie land to water.
Question:-9(a)
Write a note on pyramid of energy.
Answer:
Pyramid of Energy
The pyramid of energy is a graphical representation of the flow of energy through different trophic levels in an ecosystem. It illustrates how energy is transferred from producers to consumers and reflects the efficiency of energy transfer in a food chain or web.
Key Features:
-
Structure:
- Base: Comprises producers (e.g., plants, algae), which capture solar energy via photosynthesis, forming the largest energy reservoir.
- Middle Levels: Primary consumers (herbivores), secondary consumers (carnivores), and tertiary consumers (top predators) occupy successive levels.
- Top: Apex predators or decomposers, with the least energy.
-
Energy Flow:
- Energy enters ecosystems primarily as sunlight, converted into chemical energy by producers.
- As energy moves up trophic levels (e.g., from plants to herbivores to carnivores), only about 10% is transferred, according to the 10% Rule. The rest is lost as heat (due to the Second Law of Thermodynamics), used in metabolism, or remains unassimilated.
-
Shape:
- Always upright, as energy decreases with each trophic level. For example, a grassland ecosystem might have 10,000 kcal/m²/year at the producer level, 1,000 kcal at herbivores, 100 kcal at primary carnivores, and 10 kcal at top predators.
-
Significance:
- Demonstrates energy inefficiency in ecosystems, explaining why food chains rarely exceed 4-5 levels.
- Highlights the dependence of all organisms on producers and the finite nature of energy resources.
Example:
In a lake ecosystem:
- Producers (phytoplankton): 20,000 kcal/m²/year.
- Primary Consumers (zooplankton): 2,000 kcal.
- Secondary Consumers (small fish): 200 kcal.
- Tertiary Consumers (large fish): 20 kcal.
Ecological Importance:
The pyramid of energy underscores the need for a broad producer base to support higher trophic levels, informing conservation efforts and sustainable resource management. Unlike pyramids of numbers or biomass, it’s unaffected by organism size or seasonal variations, making it a reliable measure of ecosystem energy dynamics.
Question:-9(b)
Discuss the vegetation pattern of Western Himalayas?
Answer:
The Western Himalayas, spanning regions like Jammu and Kashmir, Himachal Pradesh, and Uttarakhand in India, exhibit a diverse vegetation pattern influenced by altitude, climate, topography, and precipitation. This region’s vegetation follows a distinct vertical zonation due to the steep elevation gradient (from ~300 m to over 6,000 m), transitioning from subtropical foothills to alpine meadows near the snowline.
Vegetation Zones of the Western Himalayas
-
Subtropical Zone (300–1,500 m)
- Climate: Warm and humid summers, mild winters; rainfall 1,000–2,000 mm annually, influenced by the monsoon.
- Vegetation:
- Sal Forests: Dominant in lower foothills (e.g., Shiwalik ranges), Shorea robusta (sal) forms dense, deciduous forests with associates like Terminalia and Syzygium.
- Mixed Deciduous Forests: Include trees like Acacia catechu (khair), Dalbergia sissoo (shisham), and Bauhinia species, interspersed with shrubs and grasses.
- Scrub Vegetation: In drier areas, thorny shrubs like Ziziphus and Carissa thrive.
- Key Features: Evergreen and deciduous mix, heavily exploited for timber and agriculture (e.g., tea plantations in lower slopes).
-
Temperate Zone (1,500–3,000 m)
- Climate: Cooler, with moderate summers and cold winters; snowfall common; rainfall decreases westward.
- Vegetation:
- Oak Forests: Quercus species (e.g., Q. leucotrichophora, banj oak) dominate, forming dense canopies with rhododendrons and laurels as undergrowth.
- Coniferous Forests: Higher up (2,000–3,000 m), Pinus roxburghii (chir pine) forms extensive stands, adapted to drier slopes. Cedrus deodara (deodar cedar) appears in moister areas, prized for its timber.
- Mixed Broadleaf Forests: Maple (Acer), horse chestnut (Aesculus), and walnut (Juglans regia) mix with conifers.
- Key Features: Rich biodiversity, including medicinal plants (e.g., Aconitum) and epiphytes; supports fauna like Himalayan black bears and leopards.
-
Subalpine Zone (3,000–4,000 m)
- Climate: Harsh, with short summers and heavy snowfall; transitional between temperate and alpine zones.
- Vegetation:
- Coniferous Dominance: Abies pindrow (silver fir) and Picea smithiana (spruce) form dense forests, often with Betula utilis (Himalayan birch) near the treeline.
- Shrubs and Undergrowth: Rhododendron campanulatum and Juniperus species form thickets, with herbaceous plants like Primula and Potentilla.
- Key Features: Stunted tree growth due to wind and cold; marks the upper limit of tree cover (treeline ~3,500–4,000 m).
-
Alpine Zone (4,000–5,500 m)
- Climate: Extremely cold, with long winters, high winds, and minimal precipitation (mostly snow); short growing season (2–3 months).
- Vegetation:
- Alpine Meadows: Above the treeline, lush grasslands (bugyals) bloom in summer with herbs like Saussurea, Anemone, and Gentiana, supporting grazing by yak and sheep.
- Dwarf Shrubs: Rhododendron anthopogon and Juniperus recurva grow low to resist wind.
- Cushion Plants and Lichens: Near snowlines, plants like Arenaria and Saxifraga adapt to rocky, nutrient-poor soils.
- Key Features: Low stature, high adaptation to UV radiation and frost; biodiversity decreases with altitude.
-
Nival Zone (>5,500 m)
- Climate: Perpetual snow and ice, extreme cold, and negligible growing season.
- Vegetation: Virtually absent, limited to sparse lichens and mosses in sheltered microhabitats.
- Key Features: Dominated by glaciers and rocky outcrops; life is minimal, mostly microbial.
Influencing Factors
- Altitude: Primary driver of zonation, with temperature dropping ~6.5°C per 1,000 m rise, shifting vegetation from broadleaf to coniferous to herbaceous.
- Precipitation: Varies from wet eastern slopes (e.g., Uttarakhand) to drier western areas (e.g., Ladakh), affecting forest density and species composition.
- Aspect: South-facing slopes are warmer and support denser forests (e.g., pine), while north-facing slopes retain moisture and favor oaks or firs.
- Soil: Thin, rocky soils at higher altitudes contrast with fertile alluvial soils in foothills, influencing plant types.
- Human Impact: Deforestation, grazing, and tourism have degraded lower forests and meadows, though conservation efforts (e.g., national parks like Jim Corbett or Nanda Devi) aim to protect them.
Ecological Significance
The Western Himalayas’ vegetation supports diverse fauna (e.g., snow leopards, musk deer), acts as a carbon sink, and provides resources like timber, fodder, and medicinal herbs. Its gradients also make it a hotspot for studying ecological succession and climate change impacts, as warming shifts vegetation zones upward.
Question:-10
Write short notes on: i) Importance of endemics ii) Xerarch iii) Systema Naturae iv) Therophytes
Answer:
i) Importance of Endemics
- Definition: Endemic species are plants or animals native and restricted to a specific geographic region (e.g., Himalayan blue poppy in the Western Himalayas).
- Importance:
- Biodiversity: Indicate unique evolutionary processes and high local adaptation, enriching global biodiversity.
- Ecosystem Role: Often keystone species, critical to local food webs or pollination (e.g., endemic bees).
- Conservation: Vulnerable to extinction due to limited range; their loss signals habitat degradation.
- Scientific Value: Offer insights into speciation and biogeography.
- Example: The Kangaroo Paw (Anigozanthos) in Western Australia supports local pollinators and reflects regional ecological history.
ii) Xerarch
- Definition: Xerarch succession is the ecological process where plant communities develop on dry, barren land (e.g., rock outcrops, sand dunes) toward a stable climax community.
- Stages:
- Pioneer Stage: Lichens and mosses colonize bare surfaces, breaking down rock and forming soil.
- Intermediate Stages: Grasses, shrubs, and drought-resistant plants (e.g., cacti) establish as soil deepens.
- Climax Stage: Depending on climate, may develop into forests or grasslands.
- Key Features: Occurs in xeric (dry) conditions; soil formation and moisture retention drive progression.
- Example: Succession on a desert rock eventually forming sparse shrubland.
iii) Systema Naturae
- Definition: Systema Naturae is a foundational work by Carl Linnaeus, first published in 1735, introducing binomial nomenclature and taxonomic classification.
- Significance:
- Classification: Organized living organisms into hierarchical groups (kingdom, class, order, genus, species).
- Nomenclature: Standardized two-part naming (e.g., Homo sapiens), still used today.
- Editions: Expanded over time; the 10th edition (1758) is the starting point for modern zoological nomenclature.
- Impact: Laid the groundwork for modern taxonomy, enabling systematic study of biodiversity.
- Example: Classified plants (e.g., Rosa canina) and animals based on shared traits.
iv) Therophytes
- Definition: Therophytes are annual plants that survive unfavorable seasons (e.g., drought, cold) as seeds, completing their life cycle in one growing season.
- Characteristics:
- Adaptation: Seed dormancy ensures survival in harsh climates (e.g., deserts, tundras).
- Growth: Rapid germination and reproduction when conditions improve.
- Examples: Wildflowers like Arabidopsis or desert annuals like poppies.
- Ecological Role: Pioneer species in disturbed or seasonal habitats; contribute to soil stabilization and early succession.
- Classification: Part of Raunkiaer’s life-form system, emphasizing survival strategy.