BBYCT-137 Solved Assignment
- Define the following terms:
i) Matric potential
ii) Holochrome
iii) Holozyme
iv) Heterocyst
v) Fluorescence - How enzyme activity gets altered by inhibitors? Explain.
- Describe the biochemistry of ammonia assimilation in plants.
- What are photosynthetic and non-photosynthetic pigments? Discuss their distribution and role in plant kingdom.
- Describe the role that macro and micro nutrients play in the growth and development of plants. Describe the deficiency symptoms of various elements in plants.
- Describe the role of auxin and cytokinin in plant development.
- a) Describe the Munch Mass Flow model of translocation of solutes.
b) Discuss the ‘sink’ to ‘source’ transition during phloem unloading. - a) Distinguish between short day and long day plants giving two examples of each. What is the role of phytochrome in flowering?
b) What are allosteric enzymes? Discuss with the help of an example. - a) What are the different biochemical and morphological changes recorded in plants in response to stress conditions? Discuss.
b) How does ABA act as a stress hormone? - a) Describe the structure of mycorrhiza. Comment on the role of mycorrhizal root association in mineral nutrition.
b) Describe the process of glycolysis with the help of a labelled diagram.
Answer:
Question:-1
Define the following terms:
i) Matric potential
ii) Holochrome
iii) Holozyme
iv) Heterocyst
v) Fluorescence
ii) Holochrome
iii) Holozyme
iv) Heterocyst
v) Fluorescence
Answer:
i) Matric potential: The component of water potential due to the adhesion of water molecules to solid surfaces (e.g., soil particles or cell walls) and capillary forces in porous media. It reflects the energy required to extract water from these surfaces and is typically negative, indicating a reduction in water availability.
ii) Holochrome: A complex of a protein and a chromophore (light-absorbing molecule) that functions as a light-harvesting unit, often in photosynthetic organisms. For example, in cyanobacteria, holochromes are associated with phycobiliproteins that capture light energy.
iii) Holozyme: The complete, catalytically active form of an enzyme, consisting of the apoenzyme (protein component) and its cofactor or coenzyme (non-protein component). The holozyme is fully functional, unlike the inactive apoenzyme alone.
iv) Heterocyst: A specialized, nitrogen-fixing cell found in certain filamentous cyanobacteria. Heterocysts have thick walls to create an anaerobic environment, protecting the nitrogenase enzyme from oxygen, enabling nitrogen fixation to convert N₂ into ammonia.
v) Fluorescence: The emission of light by a substance (fluorophore) after absorbing light or other electromagnetic radiation. The emitted light typically has a longer wavelength (lower energy) than the absorbed light, often observed in biological systems like chlorophyll or fluorescent proteins.
Question:-2
How enzyme activity gets altered by inhibitors? Explain.
Answer:
Enzyme activity is critical for catalyzing biochemical reactions, and inhibitors are molecules that reduce or block this activity. Inhibitors alter enzyme function by interacting with the enzyme or its substrate, impacting reaction rates and biological processes. This response provides a comprehensive 800-word explanation of how enzyme activity is altered by inhibitors, organized into five key sections with detailed explanations under each heading, followed by a conclusion.
1. Enzyme Function and the Role of Inhibitors
Enzymes are biological catalysts that accelerate chemical reactions by lowering the activation energy required for the reaction to proceed. They achieve this through an active site, a specific region where substrates bind to form an enzyme-substrate complex, leading to product formation. Enzyme activity depends on factors like substrate concentration, pH, temperature, and the presence of inhibitors. Inhibitors are molecules that bind to enzymes, reducing their catalytic efficiency or completely halting activity. They can be naturally occurring (e.g., metabolic regulators) or synthetic (e.g., drugs like aspirin). By interfering with substrate binding or catalysis, inhibitors modulate enzyme activity, impacting metabolic pathways, cellular processes, or disease states. Inhibitors are classified based on their binding mechanism and effect, with the primary types being competitive, non-competitive, uncompetitive, and mixed inhibitors.
2. Competitive Inhibition
Competitive inhibitors bind to the enzyme’s active site, directly competing with the substrate for access. Structurally, these inhibitors often resemble the substrate, allowing them to fit into the active site and block substrate binding. This interaction reduces the enzyme’s ability to form the enzyme-substrate complex, decreasing the reaction rate. The extent of inhibition depends on the relative concentrations of substrate and inhibitor. High substrate concentrations can outcompete the inhibitor, reducing its effect and potentially restoring maximum reaction velocity (Vmax), though the apparent affinity of the enzyme for the substrate (Km) increases, indicating a need for more substrate to achieve half Vmax. An example is methanol poisoning, where ethanol acts as a competitive inhibitor of alcohol dehydrogenase, preventing the metabolism of toxic methanol. Competitive inhibition is typically reversible, as the inhibitor can dissociate from the active site, allowing normal enzyme function to resume.
3. Non-Competitive Inhibition
Non-competitive inhibitors bind to a site on the enzyme distinct from the active site, known as an allosteric site. This binding induces a conformational change in the enzyme, reducing its catalytic efficiency without affecting substrate binding to the active site. As a result, the enzyme can still bind substrate, but the rate of product formation is diminished. In non-competitive inhibition, Vmax decreases because fewer enzyme molecules are catalytically active, while Km remains unchanged since substrate binding affinity is unaffected. This type of inhibition is often irreversible or slowly reversible, as the inhibitor’s binding to the allosteric site does not depend on substrate presence. Heavy metals like lead or mercury can act as non-competitive inhibitors by binding to sulfhydryl groups on enzymes, disrupting their structure. Non-competitive inhibition is significant in metabolic regulation, where feedback mechanisms use inhibitors to control pathway activity.
4. Uncompetitive Inhibition
Uncompetitive inhibitors bind only to the enzyme-substrate complex, not the free enzyme. This binding stabilizes the complex, preventing the release of products and slowing the reaction. Unlike competitive inhibition, uncompetitive inhibition is more pronounced at high substrate concentrations because more enzyme-substrate complexes are available for the inhibitor to bind. This results in a decrease in both Vmax and Km. The reduction in Km occurs because the inhibitor effectively removes active enzyme-substrate complexes, increasing the apparent affinity of the remaining enzyme for substrate. Uncompetitive inhibition is less common but significant in certain contexts, such as the action of drugs like lithium on inositol monophosphatase in bipolar disorder treatment. The inhibitor’s dependence on the enzyme-substrate complex makes this mechanism unique, as it does not compete with substrate but rather locks the enzyme in an inactive state after substrate binding.
5. Mixed Inhibition and Irreversible Inhibition
Mixed inhibition combines elements of competitive and non-competitive inhibition. Mixed inhibitors bind to an allosteric site but can interact with both the free enzyme and the enzyme-substrate complex, though with different affinities. This binding alters the enzyme’s conformation, affecting both substrate binding and catalysis. As a result, mixed inhibition typically increases Km (reduced substrate affinity) and decreases Vmax (reduced catalytic rate). The degree of inhibition depends on the inhibitor’s preference for the free enzyme or the complex. Irreversible inhibition, a distinct category, involves inhibitors that permanently inactivate the enzyme, often through covalent bonding to the active site or critical residues. Examples include penicillin, which irreversibly inhibits bacterial transpeptidase, and nerve agents like sarin, which bind to acetylcholinesterase. Irreversible inhibitors cause a complete loss of enzyme activity, requiring new enzyme synthesis to restore function, making them potent but potentially toxic.
Conclusion
Inhibitors play a crucial role in modulating enzyme activity by targeting specific aspects of enzyme function, such as substrate binding or catalytic efficiency. Competitive inhibitors block the active site, non-competitive inhibitors alter enzyme conformation via allosteric binding, uncompetitive inhibitors stabilize the enzyme-substrate complex, and mixed inhibitors affect both binding and catalysis. Irreversible inhibitors permanently disable enzymes, with significant implications for biological systems and medical applications. Understanding these mechanisms is vital for fields like pharmacology, where inhibitors are designed as drugs, and metabolic regulation, where they control biochemical pathways. By altering enzyme activity, inhibitors provide precise control over cellular processes, highlighting their importance in both natural and therapeutic contexts. The study of enzyme inhibition continues to advance, offering insights into disease treatment and biochemical regulation.
Question:-3
Describe the biochemistry of ammonia assimilation in plants.
Answer:
Ammonia assimilation in plants is a critical biochemical process that enables the incorporation of inorganic nitrogen into organic compounds, supporting growth and metabolism. Plants primarily acquire nitrogen in the form of nitrate or ammonium, which is converted to ammonia before being assimilated into amino acids and other nitrogen-containing molecules. This 800-word response details the biochemistry of ammonia assimilation in plants, organized into five key sections with detailed explanations, followed by a conclusion.
1. Nitrogen Acquisition and Ammonia Formation
Nitrogen is an essential element for plants, forming the backbone of amino acids, nucleic acids, and chlorophyll. Plants absorb nitrogen primarily as nitrate (NO₃⁻) from the soil, which is reduced to ammonia (NH₃) through a two-step process. First, nitrate reductase in the cytoplasm reduces nitrate to nitrite (NO₂⁻) using NADH or NADPH as an electron donor. Nitrite is then transported to chloroplasts (in leaves) or plastids (in roots), where nitrite reductase further reduces it to ammonia using ferredoxin as an electron source. Alternatively, some plants, especially those in acidic or waterlogged soils, directly uptake ammonium (NH₄⁺), which dissociates into ammonia in the cytoplasm. Ammonia, whether derived from nitrate reduction or ammonium uptake, is toxic at high concentrations and must be rapidly assimilated into organic compounds to prevent cellular damage. This assimilation occurs primarily in plastids, where enzymes facilitate the incorporation of ammonia into carbon skeletons.
2. The Glutamine Synthetase/Glutamate Synthase Cycle
The primary pathway for ammonia assimilation in plants is the glutamine synthetase/glutamate synthase (GS/GOGAT) cycle, a highly efficient two-enzyme system. Glutamine synthetase (GS) catalyzes the ATP-dependent addition of ammonia to glutamate, forming glutamine. This reaction incorporates ammonia into an organic molecule, detoxifying it and creating a nitrogen-rich compound. GS exists in two isoforms: GS1 in the cytoplasm and GS2 in chloroplasts, with GS2 being predominant in photosynthetic tissues. The glutamine produced is then used by glutamate synthase (GOGAT), which transfers the amide group from glutamine to 2-oxoglutarate, producing two molecules of glutamate. GOGAT also has two isoforms: ferredoxin-dependent GOGAT (Fd-GOGAT) in chloroplasts and NADH-dependent GOGAT (NADH-GOGAT) in plastids of non-photosynthetic tissues. The GS/GOGAT cycle is energy-intensive, requiring ATP and reducing power, but it efficiently assimilates ammonia while regenerating glutamate, which serves as a substrate for further ammonia assimilation or other biosynthetic pathways.
3. Alternative Pathways for Ammonia Assimilation
While the GS/GOGAT cycle is the dominant pathway, alternative enzymes can assimilate ammonia under specific conditions. Glutamate dehydrogenase (GDH) catalyzes the reversible incorporation of ammonia into 2-oxoglutarate to form glutamate, using NADH or NADPH as cofactors. Unlike the GS/GOGAT cycle, GDH has a lower affinity for ammonia and is primarily active when ammonia concentrations are high, such as during stress or senescence. GDH may also function in glutamate catabolism, releasing ammonia rather than assimilating it, depending on cellular conditions. Another enzyme, asparagine synthetase, incorporates ammonia into aspartate to form asparagine, particularly in roots or during nitrogen transport. These alternative pathways are less significant than the GS/GOGAT cycle but provide flexibility in nitrogen metabolism, especially in environments with fluctuating ammonia availability or during specific developmental stages.
4. Regulation of Ammonia Assimilation
Ammonia assimilation is tightly regulated to balance nitrogen use with plant growth and environmental conditions. The GS/GOGAT cycle is controlled at transcriptional, post-transcriptional, and enzymatic levels. Light and carbon availability enhance GS2 and Fd-GOGAT expression in photosynthetic tissues, linking ammonia assimilation to photosynthesis, which provides ATP and reducing power. Nitrate and ammonium levels also regulate enzyme activity; for example, high ammonium induces GS1 expression in roots. Post-translational modifications, such as phosphorylation of GS, modulate enzyme activity in response to metabolic demands. Additionally, 2-oxoglutarate availability, derived from the tricarboxylic acid (TCA) cycle, is a key regulatory factor, as it serves as a carbon skeleton for glutamate synthesis. Feedback inhibition by downstream products like glutamine and amino acids prevents excessive nitrogen assimilation, ensuring efficient resource use. This regulation integrates ammonia assimilation with carbon metabolism, optimizing plant growth.
5. Physiological and Ecological Significance
Ammonia assimilation is vital for plant physiology and ecological adaptation. By converting inorganic nitrogen into organic compounds, it supports the synthesis of amino acids, proteins, and nucleotides, which are essential for growth, reproduction, and stress responses. The GS/GOGAT cycle’s efficiency allows plants to thrive in nitrogen-limited environments, a common challenge in natural ecosystems. In legumes, ammonia assimilation is critical for symbiotic nitrogen fixation, where rhizobia in root nodules convert atmospheric nitrogen (N₂) into ammonia, which is assimilated via the GS/GOGAT cycle. This process enhances soil fertility and reduces the need for synthetic fertilizers. Ammonia assimilation also influences plant responses to environmental stresses, such as drought or salinity, by maintaining nitrogen homeostasis. Ecologically, plants with efficient ammonia assimilation contribute to nitrogen cycling, impacting soil microbial communities and ecosystem productivity.
Conclusion
Ammonia assimilation in plants is a cornerstone of nitrogen metabolism, enabling the conversion of inorganic nitrogen into organic molecules essential for growth and survival. The GS/GOGAT cycle, supported by alternative pathways like GDH, ensures efficient ammonia incorporation, while tight regulation aligns this process with environmental and metabolic cues. The biochemical machinery of ammonia assimilation, centered in plastids and linked to photosynthesis and carbon metabolism, underscores its physiological importance. Ecologically, it supports nitrogen cycling and plant adaptation to diverse habitats, particularly in nitrogen-scarce environments. Understanding these processes enhances agricultural practices, such as optimizing fertilizer use and improving crop resilience, highlighting the significance of ammonia assimilation in both natural and managed ecosystems.
Question:-4
What are photosynthetic and non-photosynthetic pigments? Discuss their distribution and role in plant kingdom.
Answer:
Photosynthetic and non-photosynthetic pigments are vital molecules in plants, contributing to light absorption, energy conversion, and various physiological functions. Photosynthetic pigments capture light for photosynthesis, while non-photosynthetic pigments serve protective, signaling, and structural roles. This 800-word response explores the nature, distribution, and roles of these pigments in the plant kingdom, organized into five sections with detailed explanations, followed by a conclusion.
1. Definition and Types of Photosynthetic Pigments
Photosynthetic pigments are light-absorbing molecules that capture solar energy to drive photosynthesis, the process by which plants convert light energy into chemical energy stored in glucose. These pigments are primarily located in the chloroplasts, specifically within the thylakoid membranes. The main photosynthetic pigments in plants are chlorophylls (chlorophyll a and b), which absorb blue and red light, reflecting green, giving plants their characteristic color. Carotenoids, including carotenes and xanthophylls, are accessory pigments that absorb blue-green light and transfer energy to chlorophylls. Phycobilins, found in algae and some cyanobacteria, absorb green and orange light, aiding photosynthesis in aquatic environments. These pigments work together to maximize light absorption across the visible spectrum, ensuring efficient energy capture for the light-dependent reactions of photosynthesis.
2. Definition and Types of Non-Photosynthetic Pigments
Non-photosynthetic pigments do not directly participate in photosynthesis but play diverse roles in plant physiology, protection, and signaling. These pigments are found in various plant tissues, including leaves, flowers, fruits, and roots. Anthocyanins, a class of flavonoids, produce red, purple, or blue colors in flowers, fruits, and leaves, often in response to environmental cues. Betalains, found in certain plants like beets and cacti, produce red-violet (betacyanins) or yellow (betaxanthins) hues, replacing anthocyanins in some species. Flavonoids, including flavones and flavonols, contribute to pale yellow or ivory colors and UV protection. Phenolic compounds and other secondary metabolites may also act as pigments, contributing to coloration and defense. Unlike photosynthetic pigments, non-photosynthetic pigments are not restricted to chloroplasts and are often synthesized in the cytoplasm or vacuoles.
3. Distribution of Photosynthetic Pigments in the Plant Kingdom
Photosynthetic pigments are widely distributed across the plant kingdom, reflecting adaptations to diverse light environments. Chlorophylls are ubiquitous in green plants, including bryophytes, ferns, gymnosperms, and angiosperms, concentrated in leaf chloroplasts to optimize photosynthesis. Carotenoids are also universal, present in all photosynthetic organisms, including algae, where they enhance light harvesting in low-light conditions, such as deep water. Phycobilins are primarily found in red algae, cyanobacteria, and some cryptophytes, enabling efficient photosynthesis in aquatic habitats where green light predominates. In higher plants, chlorophyll a is the primary pigment, with chlorophyll b and carotenoids playing accessory roles. The distribution of these pigments varies with tissue type and environmental conditions; for example, shade-adapted plants may have higher chlorophyll b levels to capture diffuse light, while sun-exposed plants increase carotenoid content for photoprotection.
4. Distribution of Non-Photosynthetic Pigments in the Plant Kingdom
Non-photosynthetic pigments are distributed across various plant groups, with their presence and concentration influenced by ecological and physiological factors. Anthocyanins are widespread in angiosperms, occurring in flowers (e.g., roses), fruits (e.g., berries), and leaves, especially during autumn senescence or stress. They are less common in gymnosperms and rare in lower plants like mosses. Betalains are restricted to the Caryophyllales order (e.g., beets, bougainvillea), where they functionally replace anthocyanins. Flavonoids are ubiquitous, found in nearly all vascular plants, contributing to coloration in petals and UV protection in leaves. These pigments are often localized in vacuoles or epidermal cells, with their expression regulated by environmental factors like light, temperature, and nutrient stress. For instance, anthocyanin accumulation in leaves increases under high light or cold stress, while betalains in desert plants aid survival in arid conditions.
5. Roles of Photosynthetic and Non-Photosynthetic Pigments
Photosynthetic pigments are essential for capturing light energy and facilitating electron transfer in photosynthesis. Chlorophyll a is the primary reaction center pigment in photosystems I and II, converting light energy into chemical energy. Chlorophyll b and carotenoids broaden the absorption spectrum, transferring absorbed energy to chlorophyll a, and protect against photooxidative damage by quenching excess energy and neutralizing reactive oxygen species. Non-photosynthetic pigments have multifaceted roles. Anthocyanins attract pollinators and seed dispersers by coloring flowers and fruits, protect against UV radiation, and act as antioxidants during stress. Betalains serve similar functions in Caryophyllales, enhancing pollination and stress tolerance. Flavonoids provide UV screening, deter herbivores, and signal symbiotic interactions, such as nitrogen-fixing bacteria in legumes. Both pigment types contribute to plant survival; photosynthetic pigments sustain primary metabolism, while non-photosynthetic pigments enhance adaptation to environmental challenges and ecological interactions.
Conclusion
Photosynthetic and non-photosynthetic pigments are integral to plant function, reflecting evolutionary adaptations to diverse environments. Photosynthetic pigments, such as chlorophylls and carotenoids, drive energy capture in photosynthesis, distributed across chloroplasts in green plants and algae. Non-photosynthetic pigments, including anthocyanins and betalains, support protection, signaling, and ecological interactions, with varied distribution in vascular plants. Their roles extend beyond aesthetics, influencing plant survival, reproduction, and stress responses. Understanding these pigments’ biochemistry and distribution enhances insights into plant physiology and informs agricultural practices, such as breeding for stress-resistant or visually appealing crops. The interplay of these pigments underscores the complexity of plant adaptation in the natural world.
Question:-5
Describe the role that macro and micro nutrients play in the growth and development of plants. Describe the deficiency symptoms of various elements in plants.
Answer:
Nutrients are essential for plant growth and development, providing the building blocks for structural components, metabolic processes, and physiological functions. Macronutrients are required in large quantities, while micronutrients are needed in trace amounts. Both are critical, and their deficiencies lead to distinct symptoms. This 800-word response explores the roles of macro and micronutrients in plants and describes deficiency symptoms, organized into five sections with detailed explanations, followed by a conclusion.
1. Role of Macronutrients in Plant Growth
Macronutrients, including nitrogen (N), phosphorus (P), potassium (K), calcium (Ca), magnesium (Mg), and sulfur (S), are vital for plant structure and metabolism. Nitrogen is a key component of amino acids, proteins, nucleic acids, and chlorophyll, driving vegetative growth and photosynthesis. Phosphorus supports energy transfer as part of ATP and phospholipids, essential for root development and flowering. Potassium regulates stomatal function, enzyme activation, and osmotic balance, enhancing water uptake and stress resistance. Calcium strengthens cell walls by forming calcium pectate, supports membrane stability, and facilitates cell signaling. Magnesium is central to chlorophyll, enabling photosynthesis, and activates enzymes in carbohydrate metabolism. Sulfur is integral to amino acids (cysteine, methionine) and coenzymes, contributing to protein synthesis and stress defense. These nutrients collectively ensure robust growth, reproduction, and resilience.
2. Role of Micronutrients in Plant Growth
Micronutrients, such as iron (Fe), manganese (Mn), zinc (Zn), copper (Cu), boron (B), molybdenum (Mo), and chlorine (Cl), are required in small amounts but are equally critical. Iron is essential for chlorophyll synthesis and acts as a cofactor in electron transport during photosynthesis and respiration. Manganese activates enzymes in photosynthesis and protects against oxidative stress. Zinc supports enzyme function, protein synthesis, and hormone (auxin) production, influencing growth regulation. Copper is involved in lignin synthesis and photosynthetic electron transport. Boron maintains cell wall integrity and supports pollen tube growth, aiding reproduction. Molybdenum is crucial for nitrogen fixation and nitrate reduction, enabling nitrogen metabolism. Chlorine contributes to photosynthesis and osmotic regulation. Though needed in trace amounts, micronutrients are indispensable for metabolic efficiency and developmental processes.
3. Macronutrient Deficiency Symptoms
Deficiency symptoms of macronutrients are often visible and disrupt plant growth. Nitrogen deficiency causes chlorosis (yellowing) in older leaves due to reduced chlorophyll, stunted growth, and poor protein synthesis, as nitrogen is mobilized to younger tissues. Phosphorus deficiency results in dark green or purplish leaves, delayed flowering, and weak root systems, reflecting impaired energy transfer. Potassium deficiency leads to marginal leaf scorching or necrosis, weak stems, and reduced disease resistance, as potassium is critical for osmotic balance. Calcium deficiency manifests as distorted young leaves, blossom-end rot in fruits (e.g., tomatoes), and weak cell walls, due to its role in structural integrity. Magnesium deficiency causes interveinal chlorosis in older leaves, as magnesium is redistributed to new growth, impairing photosynthesis. Sulfur deficiency results in pale green or yellow young leaves and stunted growth, resembling nitrogen deficiency but affecting newer tissues first.
4. Micronutrient Deficiency Symptoms
Micronutrient deficiencies produce specific symptoms, often affecting metabolic or structural functions. Iron deficiency causes interveinal chlorosis in young leaves, as iron is immobile and essential for chlorophyll synthesis. Manganese deficiency leads to chlorotic spots or mottling between veins in young leaves, impairing photosynthesis and enzyme activity. Zinc deficiency results in small, distorted leaves (rosetting), internode shortening, and reduced fruit set, due to its role in growth hormones. Copper deficiency causes wilting, leaf curling, and dieback of shoot tips, reflecting its importance in lignin and photosynthesis. Boron deficiency manifests as brittle, distorted leaves, poor pollen viability, and “hollow heart” in roots (e.g., beets), linked to cell wall and reproductive failures. Molybdenum deficiency, rare but significant, causes whip-like leaf distortion and pale leaves, disrupting nitrogen metabolism. Chlorine deficiency, uncommon, leads to wilting and chlorosis, affecting photosynthesis and water balance.
5. Nutrient Management and Plant Health
Effective nutrient management is crucial for optimizing plant growth and preventing deficiencies. Soil testing and foliar analysis help identify nutrient availability, guiding fertilization strategies. Macronutrients are typically supplied through organic (compost, manure) or inorganic fertilizers (e.g., NPK blends), while micronutrients may be applied via foliar sprays or soil amendments. Balanced nutrition prevents antagonistic interactions, such as excess potassium inhibiting magnesium uptake. Environmental factors, like soil pH, influence nutrient availability; for example, acidic soils reduce phosphorus solubility, while alkaline soils limit iron uptake. Deficiency symptoms serve as diagnostic tools, but early detection is key to avoid yield losses. Integrated approaches, including crop rotation and cover crops, enhance soil nutrient levels, supporting sustainable agriculture. Understanding nutrient roles and deficiency symptoms enables precise interventions, ensuring healthy plant development.
Conclusion
Macro and micronutrients are fundamental to plant growth, supporting structural, metabolic, and physiological functions. Macronutrients like nitrogen and phosphorus drive growth and energy transfer, while micronutrients like iron and boron fine-tune metabolic processes and reproduction. Deficiencies disrupt these functions, producing visible symptoms such as chlorosis, stunted growth, or reproductive failure, which vary by nutrient and plant tissue. Effective nutrient management, informed by deficiency symptoms and soil conditions, is essential for optimizing plant health and agricultural productivity. By addressing nutrient needs, growers can enhance plant resilience, improve yields, and contribute to sustainable ecosystems, underscoring the critical interplay between nutrition and plant development.
Question:-6
Describe the role of auxin and cytokinin in plant development.
Answer:
Auxin and cytokinin are pivotal plant hormones that regulate growth and development, orchestrating processes from cell division to organ formation. Their interplay governs plant architecture and responses to environmental cues. This 800-word response details the roles of auxin and cytokinin in plant development, organized into five sections with comprehensive explanations, followed by a conclusion.
1. Overview of Auxin and Cytokinin Functions
Auxin and cytokinin are key phytohormones with distinct yet complementary roles in plant development. Auxin, primarily indole-3-acetic acid (IAA), regulates cell elongation, differentiation, and tissue patterning, influencing processes like root initiation and apical dominance. It acts as a signaling molecule, directing growth in response to light (phototropism) and gravity (gravitropism). Cytokinin, including compounds like zeatin, promotes cell division, delays senescence, and enhances shoot formation. These hormones often interact antagonistically or synergistically, with their balance determining developmental outcomes. For instance, a high auxin-to-cytokinin ratio favors root development, while a higher cytokinin level promotes shoot growth. Their roles are mediated by complex signaling pathways involving receptors, transcription factors, and transport mechanisms.
2. Auxin in Growth and Morphogenesis
Auxin is a master regulator of plant morphogenesis, driving cell expansion and tissue differentiation. It promotes cell elongation by increasing cell wall plasticity through the activation of proton pumps, which acidify the cell wall, loosening it for expansion. In shoots, auxin maintains apical dominance, suppressing lateral bud growth by inhibiting cytokinin synthesis in axillary buds. In roots, auxin initiates lateral root formation and maintains the root meristem, ensuring continuous growth. Auxin gradients, established by polar transport via PIN proteins, are critical for organ patterning, such as in phyllotaxis (leaf arrangement) and vascular tissue differentiation. Auxin also mediates tropic responses; for example, in phototropism, uneven auxin distribution causes differential growth, bending the shoot toward light. Its versatility makes auxin essential for shaping plant architecture and adapting to environmental stimuli.
3. Cytokinin in Cell Division and Differentiation
Cytokinin is primarily known for stimulating cell division (cytokinesis) and promoting shoot development. It activates the cell cycle by upregulating cyclin-dependent kinases, ensuring meristematic activity in shoot apical meristems. Cytokinin enhances shoot organogenesis, counteracting auxin’s dominance in apical regions, and is crucial for forming leaves and reproductive structures. It also delays leaf senescence by maintaining chlorophyll levels and protein synthesis, extending photosynthetic capacity. In tissue culture, cytokinin is used to induce shoot regeneration, highlighting its role in cell differentiation. Cytokinin signaling, mediated by histidine kinase receptors and response regulators, modulates gene expression to sustain meristem function. Its influence extends to nutrient mobilization, as cytokinin directs resources to actively growing tissues, supporting overall plant vigor.
4. Auxin-Cytokinin Interactions in Development
The interplay between auxin and cytokinin is central to plant development, with their ratio determining cell fate and organ identity. In the shoot apical meristem, cytokinin promotes stem cell maintenance, while auxin directs cell differentiation at the meristem periphery, establishing organ boundaries. In roots, auxin sustains meristem activity, but cytokinin limits root growth by promoting cell differentiation, maintaining a balance between growth and maturation. During embryogenesis, auxin gradients define the apical-basal axis, while cytokinin supports cotyledon formation. In tissue culture, manipulating auxin and cytokinin levels induces specific developmental outcomes: high cytokinin favors shoot formation, high auxin promotes roots, and balanced levels produce callus. This interaction is regulated by mutual feedback; for example, auxin inhibits cytokinin biosynthesis, while cytokinin modulates auxin transport. Such coordination ensures precise developmental control.
5. Environmental and Practical Applications
Auxin and cytokinin mediate plant responses to environmental cues and have significant agricultural applications. Auxin drives tropic responses, enabling plants to optimize light capture (phototropism) or anchor roots (gravitropism). Cytokinin enhances stress tolerance by delaying senescence under drought or nutrient deficiency, preserving photosynthetic tissues. Both hormones influence fruit development; auxin promotes fruit set, while cytokinin supports cell division in developing fruits. In agriculture, synthetic auxins (e.g., 2,4-D) are used as herbicides or to stimulate rooting in cuttings, while cytokinins are applied to enhance crop yield and delay post-harvest senescence. Genetic engineering of auxin and cytokinin pathways has led to improved plant architecture and stress resistance. Understanding their roles enables targeted interventions, such as optimizing hormone levels to boost productivity or resilience in challenging environments.
Conclusion
Auxin and cytokinin are indispensable for plant development, shaping growth, organ formation, and environmental adaptation. Auxin drives cell elongation, root initiation, and tropic responses, while cytokinin promotes cell division, shoot development, and senescence delay. Their dynamic interplay governs meristem function, organ patterning, and developmental balance, with their ratio determining shoot versus root dominance. These hormones also mediate responses to environmental signals, enhancing plant survival and productivity. In agriculture, their manipulation offers tools for improving crop growth, yield, and stress tolerance. The intricate roles of auxin and cytokinin highlight their significance in plant biology, providing insights into developmental mechanisms and practical applications for sustainable agriculture.
Question:-7(a)
Describe the Munch Mass Flow model of translocation of solutes.
Answer:
The Munch Mass Flow model explains the translocation of solutes, primarily sugars, in plants through the phloem. Proposed by Ernst Munch, this model describes how osmotic pressure drives the movement of nutrients from source to sink tissues. This 400-word response details the model, organized into three sections with explanations, followed by a conclusion.
1. Principles of the Munch Mass Flow Model
The Munch Mass Flow model, also known as the pressure flow hypothesis, describes solute transport in the phloem as a result of osmotic pressure gradients. It posits that sugars, mainly sucrose, are actively loaded into sieve tubes at source tissues (e.g., leaves), creating a high solute concentration. This lowers the water potential, causing water to enter the sieve tubes from the xylem via osmosis, generating turgor pressure. At sink tissues (e.g., roots, fruits), sugars are unloaded, reducing solute concentration and allowing water to exit. This creates a pressure gradient that drives the mass flow of sap through the phloem, transporting nutrients efficiently over long distances. The model relies on the sieve tubes’ interconnected structure, which facilitates continuous flow.
2. Mechanisms of Solute Loading and Unloading
Solute movement begins with active loading at the source, where companion cells use proton pumps to create a proton gradient, enabling sucrose co-transport into sieve tubes via symporters. This active process, requiring ATP, concentrates sugars, lowering water potential and drawing in water, which increases turgor pressure. At sinks, sugars are unloaded either actively (via transporters) or passively (diffusion), depending on the sink’s metabolic needs. Unloading reduces solute concentration, raising water potential and causing water to exit, lowering turgor pressure. This differential pressure between source and sink drives the bulk flow of phloem sap, carrying sugars, amino acids, and hormones. The model accounts for bidirectional transport, as different sieve tubes can serve multiple sinks.
3. Applications and Limitations of the Model
The Munch model explains efficient nutrient distribution, supporting growth, storage, and reproduction. It is supported by evidence like aphid stylet experiments, which show high-pressure sap flow. However, limitations exist: the model simplifies phloem anatomy, ignoring sieve plate resistance, and assumes constant pressure gradients, which may vary with environmental factors. It also struggles to explain transport in non-sugar solutes or under stress conditions like drought. Despite these, the model remains a cornerstone of plant physiology, guiding research and agricultural practices.
Conclusion
The Munch Mass Flow model elegantly describes phloem translocation, driven by osmotic pressure gradients from source to sink. Active sugar loading and unloading create turgor differences, propelling sap flow. While widely applicable, the model has limitations in complex scenarios. Its insights remain vital for understanding plant nutrient transport and optimizing crop productivity.
Question:-7(b)
Discuss the ‘sink’ to ‘source’ transition during phloem unloading.
Answer:
The transition from sink to source during phloem unloading is a critical process in plant development, marking a shift in a tissue’s role from nutrient consumption to nutrient production. This process, primarily involving leaves, reflects changes in metabolic and physiological functions. This 400-word response explores the sink-to-source transition, organized into three sections with detailed explanations, followed by a conclusion.
1. Definition and Context of Sink and Source Tissues
In plants, sink tissues, such as young leaves, roots, and fruits, import nutrients like sucrose via phloem unloading to support growth and storage. Source tissues, typically mature leaves, produce and export sugars through photosynthesis, supplying sinks. The sink-to-source transition occurs as developing leaves mature, shifting from importing nutrients to exporting photosynthates. This transition is dynamic, driven by developmental cues and environmental factors. Phloem unloading, the process of releasing solutes from sieve tubes into sink cells, changes during this transition, reflecting altered transport mechanisms and tissue function. Understanding this shift is key to grasping nutrient allocation and plant growth.
2. Mechanisms of Phloem Unloading in Sinks
In sink tissues, phloem unloading occurs via two main pathways: symplastic (through plasmodesmata) or apoplastic (across cell membranes). Young leaves, as sinks, rely on symplastic unloading, where sucrose moves directly from sieve tubes to surrounding cells, supporting rapid growth. This process is passive, driven by concentration gradients, as sinks have high metabolic demands. Apoplastic unloading, involving active transport via membrane transporters, is less common in young leaves but occurs in some sinks like developing fruits. During the sink phase, companion cells and sink cells express sugar transporters and enzymes (e.g., invertase) to facilitate sucrose uptake and metabolism, ensuring energy for cell division and expansion. This unloading supports the sink’s role as a nutrient consumer.
3. Transition to Source Function
As leaves mature, they undergo the sink-to-source transition, typically progressing from tip to base. Photosynthetic capacity increases, producing surplus sugars that transform the leaf into a source. Phloem unloading diminishes, and loading begins, with sucrose actively transported into sieve tubes via proton-sucrose symporters for export to sinks. Plasmodesmata connectivity decreases, reducing symplastic unloading, while apoplastic loading dominates. Gene expression shifts, upregulating photosynthetic genes and downregulating sink-specific transporters. This transition, influenced by light, hormones (e.g., cytokinins), and sugar signaling, ensures efficient nutrient export, supporting plant growth and reproduction.
Conclusion
The sink-to-source transition during phloem unloading marks a leaf’s shift from nutrient importer to exporter, driven by increased photosynthesis and altered transport mechanisms. Symplastic unloading in sinks gives way to apoplastic loading in sources, reflecting metabolic and structural changes. This process optimizes resource allocation, enhancing plant development and productivity.
Question:-8(a)
Distinguish between short day and long day plants giving two examples of each. What is the role of phytochrome in flowering?
Answer:
Plants are classified as short day or long day based on their flowering response to day length, a process regulated by phytochrome. This 400-word response distinguishes between short day and long day plants, provides examples, and explains phytochrome’s role in flowering, organized into three sections with detailed explanations, followed by a conclusion.
1. Characteristics of Short Day Plants
Short day plants (SDPs) flower when the day length is shorter than a critical threshold, typically requiring long nights. This adaptation suits plants in regions where flowering aligns with specific seasons, such as autumn. SDPs need uninterrupted darkness for a minimum period to trigger flowering; light exposure during the night can inhibit it. The critical night length varies by species, but SDPs generally flower when nights exceed 10-12 hours. Examples include Chrysanthemum, which blooms in fall with shorter days, and Poinsettia, a tropical plant that flowers in winter under long nights. SDPs rely on precise photoperiodic cues to synchronize reproduction with favorable environmental conditions.
2. Characteristics of Long Day Plants
Long day plants (LDPs) flower when day length exceeds a critical threshold, typically requiring short nights. They are adapted to bloom in spring or summer when days are longer, ensuring reproductive success in temperate climates. LDPs need extended light exposure, often 12-16 hours, to initiate flowering, and short nights prevent inhibition of the flowering signal. Examples include Spinach, which flowers in summer with long days, and Wheat, a crop that blooms under extended daylight. LDPs use photoperiodism to time flowering with optimal conditions for pollination and seed development, enhancing survival and yield.
3. Role of Phytochrome in Flowering
Phytochrome, a photoreceptor protein, regulates flowering by sensing light quality and duration. It exists in two interconvertible forms: Pr (red light-absorbing) and Pfr (far-red light-absorbing). Pfr, formed under red light (daylight), promotes flowering in LDPs but inhibits it in SDPs. At night, Pfr reverts to Pr, allowing SDPs to flower if the dark period is sufficiently long. In LDPs, extended daylight maintains high Pfr levels, triggering flowering genes like CONSTANS and FLOWERING LOCUS T. In SDPs, long nights enable Pr accumulation, activating flowering. Phytochrome’s light-dependent toggling thus mediates photoperiodic control, ensuring flowering aligns with day length.
Conclusion
Short day plants like Chrysanthemum and Poinsettia flower under long nights, while long day plants like Spinach and Wheat bloom with extended days. Phytochrome orchestrates this process by sensing light, with Pfr promoting flowering in LDPs and inhibiting it in SDPs. This mechanism ensures reproductive timing, enhancing plant adaptation and agricultural productivity.
Question:-8(b)
What are allosteric enzymes? Discuss with the help of an example.
Answer:
Allosteric enzymes are critical regulators of metabolic pathways, exhibiting unique properties that allow them to modulate activity in response to specific molecules. This 400-word response defines allosteric enzymes, explains their characteristics and mechanisms, and discusses an example, organized into three sections with detailed explanations, followed by a conclusion.
1. Definition and Characteristics of Allosteric Enzymes
Allosteric enzymes are enzymes whose activity is regulated by the binding of effector molecules at sites other than the active site, known as allosteric sites. Unlike typical enzymes, which follow simple Michaelis-Menten kinetics, allosteric enzymes display sigmoidal kinetics due to cooperative binding, where substrate or effector binding at one subunit affects others. They often have multiple subunits, enabling conformational changes that enhance or inhibit catalytic activity. These enzymes act as metabolic switches, responding to cellular signals to maintain homeostasis. Allosteric regulation can be positive (activation by effectors) or negative (inhibition), making these enzymes pivotal in feedback control of biochemical pathways.
2. Mechanism of Allosteric Regulation
Allosteric regulation involves conformational changes triggered by effector binding. When an effector binds the allosteric site, it induces a structural shift in the enzyme, altering the active site’s affinity for substrates or catalytic efficiency. This is described by the concerted (MWC) model, where the enzyme exists in two states: tense (T, low activity) and relaxed (R, high activity). Positive effectors stabilize the R state, increasing activity, while negative effectors favor the T state, reducing it. Cooperative binding amplifies responses, as substrate binding to one subunit enhances binding to others. This mechanism allows allosteric enzymes to fine-tune metabolic flux, responding to cellular needs, such as nutrient availability or energy demands, with high sensitivity.
3. Example: Aspartate Transcarbamoylase (ATCase)
Aspartate transcarbamoylase (ATCase) is a classic allosteric enzyme in pyrimidine biosynthesis, catalyzing the condensation of aspartate and carbamoyl phosphate to form carbamoyl aspartate. ATCase has catalytic and regulatory subunits, with active sites on catalytic subunits and allosteric sites on regulatory ones. CTP, the end product, acts as a negative effector, binding allosteric sites to inhibit ATCase, preventing overproduction of pyrimidines. Conversely, ATP, a purine nucleotide, acts as a positive effector, activating ATCase to balance nucleotide synthesis. ATCase’s sigmoidal kinetics and feedback regulation exemplify how allosteric enzymes control metabolic pathways, ensuring efficient resource use.
Conclusion
Allosteric enzymes, like ATCase, regulate metabolism through effector-induced conformational changes, displaying cooperative, sigmoidal kinetics. Their ability to toggle between active and inactive states ensures precise control of biochemical pathways, responding to cellular signals. This regulatory mechanism is vital for metabolic balance, with ATCase illustrating its role in nucleotide synthesis, highlighting the significance of allosteric enzymes in plant and cellular physiology.
Question:-9(a)
What are the different biochemical and morphological changes recorded in plants in response to stress conditions? Discuss.
Answer:
Plants exhibit diverse biochemical and morphological changes in response to stress conditions, such as drought, salinity, or temperature extremes, to enhance survival and adaptation. This 400-word response explores these changes, organized into three sections with detailed explanations, followed by a conclusion.
1. Biochemical Changes in Response to Stress
Stress triggers significant biochemical alterations to protect cellular functions and maintain homeostasis. Plants accumulate compatible solutes, like proline, glycine betaine, and sugars, to stabilize proteins and membranes under osmotic stress from drought or salinity. Antioxidant systems are upregulated, with enzymes like superoxide dismutase and catalase neutralizing reactive oxygen species (ROS) generated during stress, preventing oxidative damage. Stress hormones, such as abscisic acid (ABA), increase, regulating stomatal closure to reduce water loss and activating stress-responsive genes. Secondary metabolites, including flavonoids and phenolics, are synthesized to enhance UV protection or deter pathogens. Protein synthesis shifts toward stress proteins, like heat shock proteins, which assist in protein folding under high temperatures, ensuring metabolic resilience.
2. Morphological Changes in Response to Stress
Morphological adaptations modify plant structure to cope with stress. Under drought, plants develop deeper, more extensive root systems to access water, while reducing leaf size to minimize transpiration. Salinity stress may lead to succulence, where leaves thicken to store water, as seen in halophytes. High temperatures can cause leaf rolling or curling to reduce surface area and heat absorption. In response to heavy metal stress, plants may exhibit stunted growth or altered root architecture to limit toxin uptake. Mechanical stress, like wind, induces shorter, thicker stems and increased lignification for structural support. These changes optimize resource use and enhance physical resilience, enabling plants to withstand adverse conditions.
3. Integration of Biochemical and Morphological Responses
Biochemical and morphological changes are interconnected, driven by signaling pathways that coordinate stress responses. For instance, ABA not only triggers biochemical changes like proline accumulation but also induces morphological adaptations such as stomatal closure. ROS signaling links antioxidant production with root growth adjustments. Gene expression changes, mediated by transcription factors, regulate both metabolic shifts and structural modifications. This integration ensures a holistic response, balancing immediate protection (e.g., antioxidant defense) with long-term adaptation (e.g., root system expansion). The synergy of these responses enhances plant survival across diverse stress conditions.
Conclusion
Plants respond to stress through biochemical changes, like solute accumulation and antioxidant production, and morphological adaptations, such as altered root systems and leaf structures. These responses, integrated via signaling pathways, protect cellular functions and optimize resource use. Understanding these changes informs strategies to enhance crop resilience, supporting agricultural sustainability in challenging environments.
Question:-9(b)
How does ABA act as a stress hormone?
Answer:
Abscisic acid (ABA) is a key plant hormone that orchestrates responses to environmental stresses, such as drought, salinity, and cold, by regulating physiological and molecular processes. This 400-word response explores how ABA functions as a stress hormone, organized into three sections with detailed explanations, followed by a conclusion.
1. ABA Synthesis and Stress-Induced Accumulation
ABA is synthesized in response to stress primarily in roots and leaves, derived from carotenoids via the mevalonate pathway. Under stress conditions like drought or salinity, environmental cues trigger increased expression of ABA biosynthesis genes, such as NCED3, leading to rapid ABA accumulation. This hormone is transported through the vascular system or synthesized locally in target tissues. ABA levels rise significantly during water deficit, signaled by reduced turgor or ionic imbalance, preparing the plant for stress mitigation. Its accumulation acts as a chemical signal, activating pathways that enhance tolerance and protect cellular integrity, making ABA a central player in stress perception and response.
2. Physiological Roles of ABA in Stress Response
ABA regulates key physiological processes to mitigate stress effects. It induces stomatal closure by binding to receptors in guard cells, activating ion channels that reduce turgor, thus limiting water loss during drought. This process involves secondary messengers like calcium and reactive oxygen species. ABA also promotes root growth to access deeper water while inhibiting shoot growth, optimizing resource allocation. It enhances the synthesis of compatible solutes, such as proline, which stabilize proteins and membranes under osmotic stress. Additionally, ABA upregulates aquaporin expression, facilitating water uptake. These actions collectively improve water use efficiency and maintain cellular homeostasis, enabling plants to survive adverse conditions.
3. Molecular Mechanisms of ABA Signaling
ABA exerts its effects through a complex signaling network. It binds to PYR/PYL/RCAR receptors, forming a complex that inhibits protein phosphatases (PP2Cs), allowing activation of SnRK2 kinases. These kinases phosphorylate transcription factors, such as ABFs, which activate stress-responsive genes involved in osmolyte synthesis, antioxidant production, and ion transport. This signaling cascade amplifies ABA’s effects, ensuring rapid and targeted responses. ABA also induces epigenetic changes, like histone modifications, to sustain stress memory, enhancing future resilience. This molecular framework underpins ABA’s role in coordinating stress adaptation at the genetic level.
Conclusion
ABA acts as a stress hormone by rapidly accumulating under adverse conditions, regulating stomatal closure, root growth, and osmolyte production, and activating stress-responsive genes via intricate signaling pathways. Its physiological and molecular actions enhance plant survival, making ABA indispensable for stress tolerance and adaptation in challenging environments.
Question:-10(a)
Describe the structure of mycorrhiza. Comment on the role of mycorrhizal root association in mineral nutrition.
Answer:
Mycorrhiza refers to the symbiotic association between fungi and plant roots, enhancing nutrient uptake and plant growth. This 400-word response describes the structure of mycorrhiza and its role in mineral nutrition, organized into three sections with detailed explanations, followed by a conclusion.
1. Structure of Mycorrhizal Associations
Mycorrhiza involves a mutualistic relationship where fungal hyphae integrate with plant root tissues. The two main types are ectomycorrhiza and endomycorrhiza (arbuscular mycorrhiza, AM). In ectomycorrhiza, fungal hyphae form a dense sheath (mantle) around the root surface, penetrating the root cortex to create a Hartig net, a network of hyphae between cortical cells, without entering cells. This structure is common in trees like pines. In AM, fungal hyphae penetrate root cortical cells, forming arbuscules—branched, tree-like structures within cells—for nutrient exchange, and vesicles for storage. AM is widespread in herbaceous plants and crops. Both types extend hyphae into the soil, increasing the root’s absorptive surface area, facilitating nutrient and water uptake.
2. Mechanisms of Mineral Nutrient Uptake
Mycorrhizal fungi enhance mineral nutrition by extending their hyphae far beyond the root zone, accessing nutrients unavailable to roots alone. The hyphae absorb minerals, particularly phosphorus, nitrogen, and micronutrients like zinc and copper, from the soil. In AM, arbuscules serve as the primary site for nutrient exchange, transferring minerals to the plant in exchange for carbon compounds (sugars) from photosynthesis. Ectomycorrhizal fungi release enzymes to break down organic matter, mobilizing nitrogen and phosphorus. The fungal network also improves soil structure, enhancing nutrient availability. This symbiotic exchange is regulated by plant and fungal signaling molecules, ensuring efficient nutrient transfer and mutual benefit.
3. Impact on Plant Mineral Nutrition
Mycorrhizal associations significantly boost plant mineral nutrition, particularly in nutrient-poor soils. Phosphorus, often immobile in soil, is a primary nutrient delivered by mycorrhiza, critical for energy transfer and growth. Nitrogen uptake is enhanced, supporting protein synthesis and metabolism. Micronutrient acquisition, such as zinc, improves enzyme function and stress resistance. Mycorrhizal plants exhibit better growth, higher yields, and increased resilience to drought and salinity due to improved nutrient and water uptake. In agriculture, mycorrhizal inoculation enhances crop productivity, reducing fertilizer needs and promoting sustainable practices, especially in degraded soils.
Conclusion
Mycorrhiza, with structures like the Hartig net in ectomycorrhiza and arbuscules in endomycorrhiza, extends root systems to enhance mineral uptake. By facilitating phosphorus, nitrogen, and micronutrient absorption, mycorrhizal associations improve plant growth and stress tolerance. Their role in mineral nutrition underscores their ecological and agricultural importance, supporting sustainable crop production.
Question:-10(b)
Describe the process of glycolysis with the help of a labelled diagram.
Answer:
Glycolysis is a fundamental metabolic pathway that breaks down glucose to produce energy in the form of ATP, occurring in the cytoplasm of cells.
1. Overview of Glycolysis
Glycolysis, derived from the Greek words for "sugar" and "splitting," is a ten-step enzymatic pathway that converts one molecule of glucose (C6H12O6) into two molecules of pyruvate (C3H4O3). It occurs in all living organisms, providing energy and intermediates for other metabolic pathways. The process is anaerobic, requiring no oxygen, and yields a net gain of two ATP molecules and two NADH molecules per glucose. Glycolysis is divided into two phases: the energy investment phase, where ATP is consumed, and the energy payoff phase, where ATP and NADH are produced. This pathway is critical for energy production, especially in low-oxygen conditions.
2. Steps of Glycolysis
The energy investment phase (steps 1-5) begins with glucose phosphorylation by hexokinase, forming glucose-6-phosphate, using one ATP. Phosphoglucose isomerase converts this to fructose-6-phosphate, which is further phosphorylated by phosphofructokinase-1, consuming another 1 ATP, yielding fructose-1,6-bisphosphate. Aldolase cleaves this into dihydroxyacetone phosphate and glyceraldehyde-3-phosphate, which are interconverted by triose phosphate isomerase. The energy payoff phase (steps 6-10) involves glyceraldehyde-3-phosphate dehydrogenase, which oxidizes glyceraldehyde-3-phosphate, producing NADH and 1,3-bisphosphoglycerate. Phosphoglycerate kinase transfers a phosphate to ADP, forming ATP and 3-phosphoglycerate. Subsequent enzymes (phosphoglycerate mutase, enolase, and pyruvate kinase) convert 3-phosphoglycerate to pyruvate, generating another ATP per triose, resulting in a net gain of two ATP and two NADH.
3. Labelled Diagram of Glycolysis
The diagram illustrates the ten steps of glycolysis, showing glucose entering the pathway, the enzymes involved, and the products (pyruvate, ATP, NADH). Key intermediates (e.g., glucose-6-phosphate, fructose-1,6-bisphosphate) and energy transactions (ATP consumption/production, NADH formation) are labelled. The energy investment phase is depicted with ATP inputs, while the payoff phase highlights ATP and NADH outputs, providing a clear visual summary of the process.
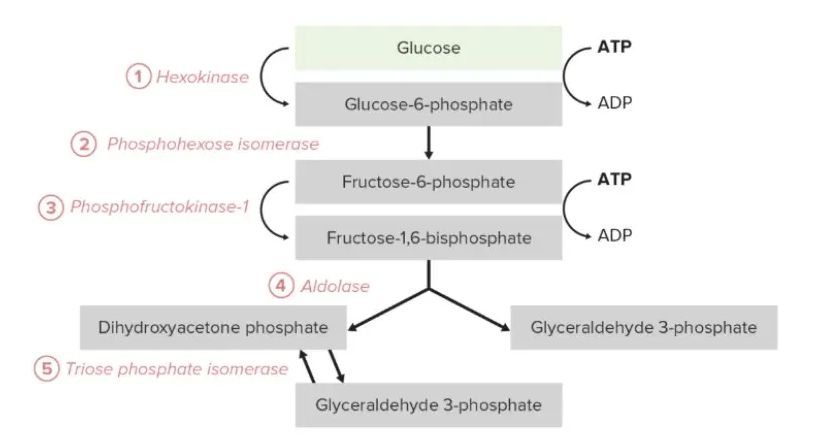
Conclusion
Glycolysis is a universal pathway that efficiently converts glucose into pyruvate, generating ATP and NADH. Its two phases balance energy investment and payoff, supporting cellular energy needs. The labelled diagram clarifies the sequence of reactions, highlighting key intermediates and energy transfers, underscoring glycolysis’s role as a cornerstone of cellular metabolism.