BBYET-143 Solved Assignment
- a) Define totipotency. Describe any two application of plant tissue culture.
b) What is a protoplast? Describe the technique of somatic hybridization with the help of a diagram. - Describe the origin, cytology and distribution of Triticum aestivum Linn.
- Describe the origin, taxonomy, morphological characters and distribution of rice.
- Write short note on Domestication syndrome and Colony hybridization.
- Describe the procedure for construction of cDNA and genomic libraries.
- a) Legumes are considered as nutritionally important foods? Explain the statement with the help of suitable examples.
b) Write a detailed note on economic importance of spices. - a) Plant growth regulators form an important component of plant tissue culture medium. Justify the statement.
b) Discuss the role of plant tissue culture in germplasm conservation. - Describe the technique of Agrobacterium-mediated transformation with the help of a diagram.
- Describe the morphology, cultivation and uses of any two fibre yielding plants.
- a) Differentiate between Northern and Western blotting.
b) Write sort note on rhizogenesis and caulogenesis.
Answer:
Question:-1(a)
Define totipotency. Describe any two application of plant tissue culture.
Answer:
Comprehensive Solution: Totipotency and Applications of Plant Tissue Culture
1. Definition of Totipotency
Totipotency refers to the inherent ability of a single plant cell to divide, differentiate, and develop into a complete, functional plant. This unique characteristic is a hallmark of plant cells, distinguishing them from most animal cells, which typically lose this capability early in development. Totipotency arises from the genetic information contained within the cell, allowing it to express all necessary genes to form various tissues and organs, such as roots, stems, leaves, and flowers. In practical terms, a totipotent cell can regenerate an entire plant under suitable environmental and nutritional conditions, typically provided in vitro through plant tissue culture techniques. This property is critical in plant biotechnology, enabling the propagation of plants from single cells or small tissue samples. For instance, meristematic cells, found in shoot and root tips, are highly totipotent, making them ideal for tissue culture applications. Understanding totipotency is foundational for leveraging plant tissue culture in agriculture, horticulture, and genetic engineering.
2. Application 1: Micropropagation
Micropropagation is a widely used application of plant tissue culture that exploits totipotency to produce large numbers of genetically identical plants (clones) from a single parent plant. This technique involves culturing small tissue explants, such as shoot tips or nodal segments, in a sterile, nutrient-rich medium containing growth regulators like auxins and cytokinins. The totipotent cells in the explant divide and differentiate to form multiple shoots, which can be rooted to develop complete plantlets. Micropropagation is particularly valuable for producing disease-free plants, especially in crops like bananas, orchids, and potatoes, where viral infections can devastate yields. It also enables rapid multiplication of elite or rare plant varieties, ensuring uniformity in traits like yield or flower quality. For example, in orchid cultivation, micropropagation has revolutionized commercial production by allowing thousands of plants to be generated from a single meristem, reducing costs and time compared to traditional seed propagation. This application enhances agricultural efficiency and supports the conservation of endangered plant species.
3. Application 2: Somatic Embryogenesis
Somatic embryogenesis is another significant application of plant tissue culture, utilizing totipotency to produce embryos from somatic (non-reproductive) cells. In this process, totipotent cells from tissues like leaves or roots are induced to form embryo-like structures in vitro, which develop into complete plants. The process begins with the culture of explants on a medium supplemented with specific hormones, such as 2,4-dichlorophenoxyacetic acid, to stimulate cell division and embryogenic callus formation. These embryos can be encapsulated to create synthetic seeds, facilitating storage and transport. Somatic embryogenesis is crucial for mass propagation of economically important crops, such as coffee, oil palm, and conifers, where seed-based propagation is slow or unreliable. It also supports genetic engineering by providing a platform for introducing desirable traits, such as pest resistance, into embryogenic cells. For instance, somatic embryogenesis in oil palm has enabled the clonal propagation of high-yielding varieties, boosting plantation productivity. This application underscores the versatility of totipotency in addressing agricultural challenges.
Conclusion
Totipotency is a cornerstone of plant tissue culture, enabling innovative applications like micropropagation and somatic embryogenesis. These techniques harness the regenerative potential of plant cells to address critical needs in agriculture, horticulture, and conservation. By facilitating the rapid production of disease-free, genetically uniform plants and supporting genetic modification, plant tissue culture continues to revolutionize plant science, ensuring sustainable crop production and biodiversity preservation.
Question:-1(b)
What is a protoplast? Describe the technique of somatic hybridization with the help of a diagram.
Answer:
Comprehensive Solution: Protoplast and Somatic Hybridization Technique
1. Definition of Protoplast
A protoplast is a plant cell from which the rigid cell wall has been removed, leaving the plasma membrane, cytoplasm, nucleus, and other organelles intact. This wall-less state makes protoplasts fragile but highly versatile for biotechnological applications. Protoplasts are typically isolated from plant tissues, such as leaves, roots, or callus, using enzymatic digestion with enzymes like cellulase and pectinase, which degrade the cell wall components. Due to their totipotent nature, protoplasts can regenerate into whole plants under appropriate culture conditions, making them valuable in plant tissue culture. Their ability to fuse with other protoplasts is key to techniques like somatic hybridization, enabling the creation of novel genetic combinations that are difficult to achieve through conventional breeding.
2. Overview of Somatic Hybridization
Somatic hybridization is a biotechnological technique that involves the fusion of protoplasts from two different plant species or varieties to produce a hybrid cell with combined genetic material. Unlike sexual hybridization, which is limited by reproductive barriers, somatic hybridization bypasses such constraints, allowing the creation of hybrids between distantly related species. The resulting hybrid cells can regenerate into whole plants, known as somatic hybrids, which may exhibit desirable traits from both parents, such as disease resistance, stress tolerance, or enhanced yield. The process is widely used in crop improvement, particularly for species where traditional breeding is challenging. The technique involves protoplast isolation, fusion, selection of hybrid cells, and regeneration of plants, each step requiring precise control of conditions.
3. Steps in Somatic Hybridization Technique
Protoplast Isolation
Protoplasts are isolated from plant tissues, typically leaves or mesophyll cells, by enzymatic digestion. The tissue is incubated in a solution containing cellulase and pectinase enzymes in a hypotonic buffer, which degrades the cell wall and releases protoplasts. The protoplasts are filtered, centrifuged, and washed to obtain a pure suspension. This step is performed under sterile conditions to prevent contamination.
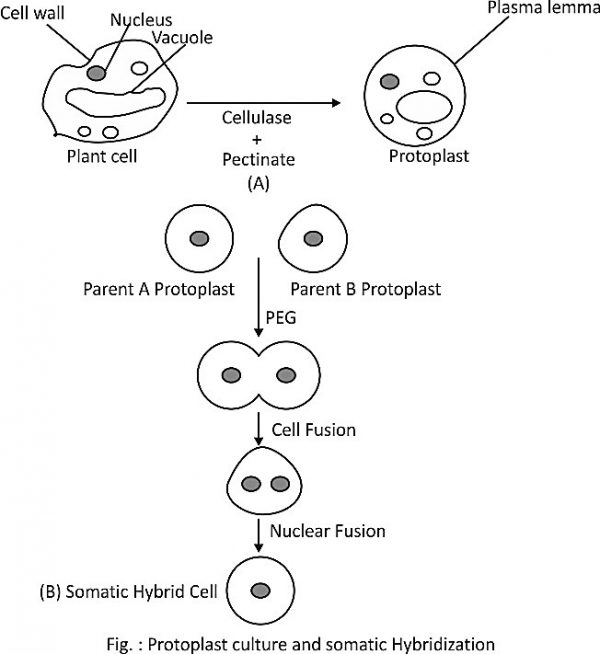
Protoplast Fusion
Fusion of protoplasts from two different plants is induced using chemical or electrical methods. Chemical fusion involves agents like polyethylene glycol (PEG), which promotes membrane adhesion and fusion by altering surface charges. Electrofusion uses an electric field to destabilize membranes, facilitating fusion. The fused protoplasts, called heterokaryons, contain nuclei and organelles from both parent cells. A diagram of this process would show two protoplasts merging, with PEG or electrodes inducing fusion, resulting in a single cell with mixed contents.
Selection of Hybrid Cells
After fusion, hybrid cells must be distinguished from unfused or homokaryotic cells. Selection methods include biochemical markers (e.g., resistance to specific antibiotics), fluorescence-based sorting, or complementation of mutant traits. For example, if one parent lacks a vital enzyme and the other has it, only hybrid cells will survive on a selective medium.
Regeneration of Somatic Hybrids
Selected hybrid cells are cultured on a nutrient-rich medium with growth regulators like auxins and cytokinins to induce cell division and form a callus. The callus is then transferred to a regeneration medium to develop shoots and roots, eventually forming a complete plant. The resulting somatic hybrid is tested for desired traits.
Conclusion
Protoplasts, as wall-less plant cells, are pivotal in somatic hybridization, a powerful technique for creating novel plant hybrids. By isolating protoplasts, inducing their fusion, selecting hybrid cells, and regenerating plants, somatic hybridization overcomes barriers of conventional breeding. This method has significant applications in agriculture, enabling the development of crops with enhanced traits, such as improved resistance or yield, contributing to food security and sustainable farming.
Question:-2
Describe the origin, cytology and distribution of Triticum aestivum Linn.
Answer:
Comprehensive Solution: Origin, Cytology, and Distribution of Triticum aestivum Linn
1. Origin of Triticum aestivum Linn
Triticum aestivum Linn, commonly known as bread wheat, is one of the most significant cereal crops globally, serving as a staple food for billions. Its origin traces back to the Fertile Crescent, a region encompassing parts of modern-day Iraq, Syria, Lebanon, Jordan, Israel, and Turkey, where agriculture began around 10,000 years ago. Bread wheat is a hexaploid species, resulting from complex hybridization events involving wild grass species. It evolved through natural and human-mediated hybridization between Triticum turgidum (tetraploid, emmer wheat) and Aegilops tauschii (diploid, goat grass). This hybridization likely occurred in the Caspian Sea region or Transcaucasia, where these species coexisted. Archaeological evidence, such as carbonized wheat grains found at sites like Çatalhöyük in Turkey, suggests that wheat cultivation spread from the Fertile Crescent to Europe, Asia, and Africa by 6,000 BCE. Domestication involved selecting traits like non-shattering spikes and larger grains, which improved yield and ease of harvest. Over millennia, human selection and migration facilitated the diversification of wheat varieties, adapting to various climates and soils, making T. aestivum a cornerstone of global agriculture.
2. Cytology of Triticum aestivum Linn
The cytology of Triticum aestivum is characterized by its hexaploid genome, which consists of 42 chromosomes (2n=6x=42). This complex genetic makeup results from its polyploid origin, combining three distinct genomes: A, B, and D. The A genome originates from Triticum urartu, the B genome likely from an ancestor related to Aegilops speltoides, and the D genome from Aegilops tauschii. Each genome contributes 14 chromosomes, organized into seven homoeologous groups, where each group contains one chromosome pair from each genome (e.g., chromosome 1A, 1B, 1D). This polyploidy enhances genetic diversity, allowing wheat to adapt to diverse environmental conditions and resist stresses like drought or disease. The large genome size, approximately 17 gigabases, contains numerous repetitive sequences and transposable elements, complicating genetic analysis but providing a reservoir of genetic variation. Cytologically, T. aestivum exhibits regular meiosis, with bivalent chromosome pairing during cell division, ensuring stable inheritance. However, its polyploid nature allows for gene redundancy, enabling tolerance to mutations and facilitating breeding for desirable traits like high yield or gluten quality. Advances in cytogenetic techniques, such as fluorescence in situ hybridization (FISH), have enabled detailed mapping of wheat chromosomes, aiding in the identification of genes controlling agronomic traits.
3. Distribution of Triticum aestivum Linn
Triticum aestivum is cultivated worldwide, reflecting its adaptability to a wide range of climates, soils, and altitudes. Its global distribution spans temperate, subtropical, and semi-arid regions, with major production areas in Asia, Europe, North America, and Australia. In Asia, countries like China, India, and Pakistan are among the largest producers, with India alone cultivating wheat across 30 million hectares, primarily in the Indo-Gangetic plains. In Europe, Russia, Ukraine, and France dominate production, leveraging fertile soils and advanced farming techniques. North America, particularly the Great Plains of the United States and Canada, produces significant quantities of hard red spring and winter wheat varieties. Australia’s wheat belt, spanning New South Wales and Western Australia, contributes to global exports. T. aestivum thrives in regions with annual rainfall of 300–1,000 mm and temperatures between 10–25°C during the growing season, though irrigation and breeding have extended its cultivation to drier areas, such as parts of the Middle East and North Africa. Altitudinally, it is grown from sea level to over 3,000 meters, as seen in the Andean highlands and Tibetan Plateau. The crop’s versatility is enhanced by breeding programs developing varieties resistant to pests, diseases, and extreme weather, ensuring its presence in diverse agroecosystems. Wheat is typically grown in rotation with other crops like maize or legumes to maintain soil fertility and reduce pest cycles.
Conclusion
Triticum aestivum Linn, or bread wheat, is a vital crop with a rich history and complex biology. Originating in the Fertile Crescent through hybridization events, its hexaploid genome, comprising A, B, and D genomes, provides genetic resilience and adaptability. This cytological complexity underpins its widespread distribution across diverse climates and regions, from the plains of India to the highlands of the Andes. As a cornerstone of global food security, T. aestivum’s cultivation supports billions, driven by its ability to thrive under varied conditions and continuous improvements through breeding. Its origin, cytology, and distribution highlight its significance in agriculture and its role in sustaining human populations worldwide.
Question:-3
Describe the origin, taxonomy, morphological characters and distribution of rice.
Answer:
Comprehensive Solution: Origin, Taxonomy, Morphological Characters, and Distribution of Rice
1. Origin of Rice
Rice, scientifically known as Oryza sativa, is one of the world’s most critical staple crops, feeding over half of the global population. Its origin is traced to the Yangtze River Valley in China, where archaeological evidence, including phytoliths and carbonized grains, suggests domestication began around 7,000–9,000 years ago. Wild rice, Oryza rufipogon, is considered the progenitor of cultivated rice, with domestication involving human selection for traits like non-shattering grains, higher yield, and uniform germination. A secondary center of origin exists in South Asia, particularly India, where Oryza indica varieties were domesticated from Oryza nivara. Genetic studies indicate two major subspecies, japonica (short-grained, sticky) and indica (long-grained, non-sticky), evolved independently due to geographic isolation. Domestication spread rice cultivation to Southeast Asia, the Middle East, and Africa by 2,000 BCE, facilitated by trade and migration. Ancient texts, such as Chinese agricultural records and Indian Vedic literature, document rice’s cultural and economic significance. Human selection transformed wild rice’s brittle rachis and low yield into modern cultivars, making rice a cornerstone of global agriculture and food security.
2. Taxonomy of Rice
Rice belongs to the genus Oryza in the family Poaceae, a diverse group of grasses that includes wheat, maize, and barley. The genus Oryza comprises approximately 22 species, with two cultivated species: Oryza sativa (Asian rice) and Oryza glaberrima (African rice). Oryza sativa is further divided into two subspecies: sativa subsp. japonica and sativa subsp. indica, distinguished by grain shape, growth habits, and ecological preferences. Japonica thrives in temperate regions, while indica is suited to tropical climates. A third group, javanica (or tropical japonica), is sometimes recognized for its intermediate traits. Taxonomically, rice is classified as follows: Kingdom: Plantae; Phylum: Tracheophyta; Class: Liliopsida; Order: Poales; Family: Poaceae; Genus: Oryza; Species: sativa. Molecular studies, including chloroplast and nuclear DNA sequencing, have clarified relationships within Oryza, revealing O. rufipogon and O. nivara as wild ancestors. The taxonomy aids breeding programs by identifying genetic resources for traits like disease resistance or drought tolerance, enhancing rice’s adaptability to diverse environments.
3. Morphological Characters of Rice
Rice is an annual grass, though some perennial varieties exist in tropical regions. It typically grows 1–1.8 meters tall, with erect or spreading culms (stems) that are hollow and jointed at nodes. The leaves are long, narrow, and lanceolate, with a prominent midrib and sheaths enveloping the stem. The ligule, a membranous structure at the leaf base, distinguishes rice from other grasses. The inflorescence is a panicle, bearing spikelets that each contain a single floret. Each spikelet has two glumes, a lemma, a palea, six stamens, and a pistil with two feathery stigmas. The grain (caryopsis) is enclosed in a husk formed by the lemma and palea, varying in color (white, brown, red, or black) and shape (short, medium, or long). Roots are fibrous, with adventitious roots supporting the plant in flooded conditions, a trait critical for paddy cultivation. Japonica grains are short and round, while indica grains are long and slender. These morphological traits, particularly grain size and panicle structure, are key for yield and market preferences, influencing breeding for high-performing varieties.
4. Distribution of Rice
Rice is cultivated across tropical, subtropical, and temperate regions, reflecting its adaptability to diverse climates and soils. Asia dominates production, accounting for over 90% of global output, with China, India, Indonesia, Bangladesh, and Vietnam as leading producers. In China, rice is grown in the Yangtze and Pearl River basins, while India cultivates it in the Gangetic plains and southern states like Andhra Pradesh. Southeast Asia, including Thailand and the Philippines, specializes in indica varieties for export. Africa grows both O. sativa and O. glaberrima, with West Africa’s Niger Delta and East Africa’s Tanzania as key regions. In the Americas, the United States (Arkansas, Louisiana) and Brazil produce rice in irrigated or rainfed systems. Europe, notably Italy and Spain, cultivates japonica for risotto varieties. Rice thrives in lowland flooded paddies, upland rainfed fields, and deepwater systems, requiring 1,000–2,500 mm of annual rainfall and temperatures of 20–35°C. Altitudinally, it ranges from sea level to 2,500 meters, as seen in the Himalayan foothills. Breeding for salinity tolerance and drought resistance has expanded cultivation to marginal lands, ensuring rice’s global presence.
Conclusion
Rice, Oryza sativa, is a vital crop with a rich history and wide-reaching impact. Originating in China and South Asia, its domestication from wild ancestors shaped global agriculture. Its taxonomy, centered on japonica and indica subspecies, guides breeding efforts. Morphologically, rice’s adaptive traits, like its panicle and root system, support its cultivation across varied ecosystems. Its distribution spans Asia, Africa, the Americas, and Europe, feeding billions and sustaining economies. As climate challenges intensify, rice’s genetic diversity and adaptability ensure its continued role in food security and cultural heritage.
Question:-4
Write short note on Domestication syndrome and Colony hybridization.
Answer:
Comprehensive Solution: Domestication Syndrome and Colony Hybridization
1. Domestication Syndrome
Domestication syndrome refers to a suite of phenotypic traits that consistently appear in plants and animals during their transition from wild to cultivated forms due to human selection. In plants, this phenomenon is observed in crops like wheat, rice, maize, and legumes, where selective pressures during domestication favor traits that enhance agricultural utility. Key traits include reduced seed shattering, increased seed size, uniform germination, reduced dormancy, and altered plant architecture. For instance, wild cereals like Oryza rufipogon (wild rice) have seeds that shatter easily to disperse naturally, but cultivated rice (Oryza sativa) has non-shattering panicles, ensuring seeds remain on the plant for harvest. Larger seeds, as seen in domesticated maize compared to its wild ancestor teosinte, improve yield and ease of processing. Uniform germination eliminates the staggered sprouting of wild plants, enabling synchronized crop cycles. Reduced dormancy ensures rapid germination under favorable conditions, critical for farming schedules. Plant architecture changes, such as shorter, more compact plants in wheat, enhance resistance to lodging (falling over) and improve harvest efficiency. These traits arise from genetic changes, often involving mutations in regulatory genes like Q in wheat or sh4 in rice, which control seed shattering. Domestication syndrome reflects human-driven evolution, prioritizing traits that align with agricultural needs, transforming wild species into reliable crops over millennia.
2. Colony Hybridization
Colony hybridization is a molecular biology technique used to identify specific DNA sequences within a large population of bacterial colonies, typically those transformed with recombinant DNA. This method is crucial in gene cloning, functional genomics, and biotechnology, enabling researchers to screen bacterial libraries for clones containing desired genes or DNA fragments. The process begins with growing bacterial colonies on an agar plate, each colony representing a clone harboring a unique DNA insert within a plasmid vector. These colonies are transferred onto a nitrocellulose or nylon membrane, creating a replica of the plate. The cells on the membrane are lysed to release their DNA, which is denatured into single strands and fixed onto the membrane. A labeled DNA probe, complementary to the target sequence, is then applied to the membrane under controlled hybridization conditions. The probe, which may be radioactively or fluorescently labeled, binds specifically to the target DNA through base-pairing. After washing away unbound probe, the membrane is visualized (e.g., via autoradiography or fluorescence) to identify colonies with the desired DNA. These positive colonies are traced back to the original agar plate for further analysis, such as DNA sequencing or gene expression studies. Colony hybridization is highly sensitive, capable of detecting rare sequences in complex libraries, and is widely used in constructing genomic or cDNA libraries, identifying disease-resistance genes in plants, or screening for transgenic organisms. Its precision and scalability make it a cornerstone of molecular biology research.
3. Applications and Significance
Domestication Syndrome Applications
Understanding domestication syndrome is vital for crop improvement and breeding programs. By identifying genes associated with domestication traits, breeders can enhance yield, quality, and resilience. For example, manipulating genes like Tb1 in maize can optimize plant architecture for modern farming. Domestication syndrome also informs efforts to domesticate new species, such as perennial grains, to create sustainable crops with traits like reduced seed shattering. Additionally, studying domestication genes aids in conserving wild relatives of crops, which serve as genetic reservoirs for traits like drought tolerance. This knowledge bridges archaeology, genetics, and agriculture, revealing how ancient human practices shaped modern food systems.
Colony Hybridization Applications
Colony hybridization has broad applications in biotechnology and plant science. It is instrumental in constructing and screening genomic libraries to isolate genes for traits like pest resistance in crops. For instance, identifying Bt genes in bacteria for transgenic crops relies on this technique. It also supports functional genomics by enabling the study of gene expression in specific tissues. In medical research, colony hybridization screens bacterial libraries for genes linked to antibiotic production. Its role in synthetic biology includes identifying engineered DNA constructs. The technique’s ability to handle large clone populations ensures efficient discovery of rare genetic elements, advancing genetic engineering and crop improvement.
Conclusion
Domestication syndrome and colony hybridization represent pivotal concepts in plant science and molecular biology. Domestication syndrome illustrates how human selection transformed wild plants into productive crops through traits like non-shattering seeds and uniform germination, shaping global agriculture. Colony hybridization, a powerful molecular tool, enables precise identification of DNA sequences, driving advancements in gene cloning and biotechnology. Together, these concepts highlight the interplay of evolutionary biology and modern technology in enhancing crop productivity and genetic research, ensuring food security and scientific innovation in an ever-changing world.
Question:-5
Describe the procedure for construction of cDNA and genomic libraries.
Answer:
Comprehensive Solution: Procedure for Construction of cDNA and Genomic Libraries
1. Overview of cDNA and Genomic Libraries
cDNA and genomic libraries are essential tools in molecular biology, used to store and study DNA sequences from an organism. A cDNA (complementary DNA) library is a collection of DNA fragments synthesized from mRNA, representing the expressed genes in a specific tissue or cell type at a given time. It contains only the coding regions (exons) of genes, excluding introns and non-coding sequences. In contrast, a genomic library encompasses the entire genomic DNA of an organism, including coding and non-coding regions, introns, exons, and regulatory elements. Both libraries are constructed by inserting DNA fragments into vectors, such as plasmids or bacteriophages, which are then introduced into host cells (typically Escherichia coli) for replication and storage. These libraries are critical for gene discovery, functional genomics, and biotechnology applications, such as identifying disease-resistance genes in plants or studying gene regulation. The construction of each library involves distinct procedures tailored to their specific purposes.
2. Procedure for Constructing a cDNA Library
RNA Extraction and mRNA Isolation
The construction of a cDNA library begins with the extraction of total RNA from a specific tissue, such as plant leaves or animal cells, using methods like TRIzol or column-based kits. Since mRNA constitutes only 1–5% of total RNA, it is purified using oligo-dT chromatography, which exploits the poly-A tail of eukaryotic mRNA to bind it to oligo-dT beads, separating it from rRNA and tRNA.
cDNA Synthesis
The purified mRNA serves as a template for cDNA synthesis. First-strand cDNA is synthesized using reverse transcriptase and an oligo-dT primer, which anneals to the poly-A tail, or random hexamer primers for broader coverage. The reaction produces a single-stranded DNA-RNA hybrid. The mRNA is then degraded using RNase H, and the second-strand cDNA is synthesized by DNA polymerase I, creating double-stranded cDNA. The ends of cDNA are often blunted using T4 DNA polymerase to facilitate ligation.
Cloning into Vectors
The double-stranded cDNA is ligated into a suitable vector, such as a plasmid or lambda phage, using DNA ligase. Vectors contain restriction sites, origins of replication, and selectable markers (e.g., antibiotic resistance genes) to ensure successful cloning. Adapters or linkers may be added to cDNA ends to match vector restriction sites. The recombinant vectors are introduced into E. coli via transformation or electroporation.
Library Storage and Screening
Transformed E. coli cells are grown on selective media, forming colonies or plaques, each containing a unique cDNA insert. These clones are pooled to create the cDNA library, which is stored as glycerol stocks at -80°C. The library can be screened using techniques like colony hybridization or PCR to identify clones with specific genes, such as those for stress tolerance in plants.
3. Procedure for Constructing a Genomic Library
Genomic DNA Extraction
Genomic library construction starts with extracting high-quality genomic DNA from an organism’s cells, such as plant leaf tissue or animal blood, using methods like CTAB (for plants) or phenol-chloroform extraction. The DNA is purified to remove proteins, polysaccharides, and other contaminants, ensuring it is suitable for enzymatic manipulation.
DNA Fragmentation
The genomic DNA, which is large and complex (e.g., ~3 billion base pairs in humans), is fragmented into manageable sizes (10–20 kb for plasmids, 30–40 kb for cosmids) to fit into vectors. Fragmentation is achieved using partial restriction enzyme digestion, which cuts DNA at specific sites, or mechanical shearing, followed by size selection via gel electrophoresis to obtain fragments of uniform length.
Cloning into Vectors
The DNA fragments are ligated into vectors, such as plasmids, cosmids, or bacterial artificial chromosomes (BACs), depending on the insert size. Vectors are chosen based on their capacity and stability; for example, BACs can carry up to 300 kb. The ligation mixture is introduced into E. coli via transformation, and transformed cells are selected using antibiotic resistance markers present in the vector.
Library Storage and Screening
The transformed E. coli cells are grown on agar plates, forming colonies or plaques, each containing a unique genomic DNA fragment. These clones are pooled to form the genomic library, stored as glycerol stocks or lyophilized cultures. Screening involves hybridization with labeled probes or PCR to identify clones with specific sequences, such as regulatory regions or entire genes.
Conclusion
cDNA and genomic libraries are indispensable for molecular biology research, offering distinct insights into gene expression and genomic organization. cDNA libraries, derived from mRNA, capture expressed genes, ideal for studying tissue-specific functions or cloning coding sequences. Genomic libraries, encompassing entire genomes, provide a comprehensive resource for analyzing gene structure, regulation, and non-coding regions. Their construction involves meticulous steps—RNA extraction, cDNA synthesis, and cloning for cDNA libraries; DNA fragmentation, ligation, and transformation for genomic libraries. These libraries enable breakthroughs in biotechnology, from developing transgenic crops to understanding genetic diseases, underscoring their role in advancing science and agriculture.
Question:-6(a)
Legumes are considered as nutritionally important foods? Explain the statement with the help of suitable examples.
Answer:
Comprehensive Solution: Nutritional Importance of Legumes
1. Nutritional Composition of Legumes
Legumes, belonging to the family Fabaceae, are nutritionally important foods due to their rich and balanced nutrient profile, making them a cornerstone of diets worldwide. They are excellent sources of plant-based protein, typically containing 20–30% protein by dry weight, which is significantly higher than most cereals. For example, soybeans provide approximately 36 g of protein per 100 g, making them a vital protein source for vegetarians and vegans. Legumes are also high in dietary fiber, both soluble and insoluble, which promotes digestive health and regulates blood sugar. Chickpeas, for instance, contain about 7 g of fiber per 100 g, aiding in cholesterol management. Additionally, legumes are rich in complex carbohydrates, providing sustained energy, and are low in fat, with exceptions like peanuts and soybeans, which contain healthy unsaturated fats. They are packed with essential micronutrients, including iron, potassium, magnesium, and B vitamins like folate. Lentils, for example, supply 90% of the daily folate requirement per 100 g, crucial for DNA synthesis and pregnancy health. This diverse nutrient profile positions legumes as a nutrient-dense food, addressing multiple dietary needs.
2. Health Benefits of Legumes
The nutritional composition of legumes translates into numerous health benefits, reinforcing their importance in human diets. Their high protein content supports muscle growth, tissue repair, and immune function, making them a cost-effective alternative to animal proteins in resource-limited regions. For instance, black beans are used in Latin American diets to meet protein needs affordably. The fiber in legumes reduces the risk of cardiovascular diseases by lowering LDL cholesterol and improves gut health by fostering beneficial microbiota. Studies show that regular consumption of kidney beans can reduce heart disease risk by 20%. Legumes have a low glycemic index, making them ideal for managing diabetes; mung beans, for example, stabilize blood sugar due to their slow-release carbohydrates. Micronutrients like iron in lentils combat anemia, particularly in developing countries, while magnesium in peas supports nerve and muscle function. Legumes also contain bioactive compounds, such as isoflavones in soybeans, which have antioxidant properties and may reduce cancer risk. These health benefits make legumes a staple in dietary guidelines globally, recommended for daily consumption in balanced diets.
3. Examples Highlighting Nutritional Importance
Specific legumes exemplify their nutritional significance across cultures and dietary contexts. Soybeans are a nutritional powerhouse, widely consumed in East Asia as tofu, tempeh, or soy milk. They provide complete proteins, containing all essential amino acids, and are rich in omega-3 fatty acids, supporting heart health. In India, lentils (Lens culinaris) are a dietary staple, consumed as dal, offering high protein and iron content, crucial for addressing malnutrition in vegetarian populations. Chickpeas (Cicer arietinum), popular in Middle Eastern dishes like hummus, supply fiber and protein, making them a favorite in plant-based diets. Peanuts (Arachis hypogaea), technically legumes, are nutrient-dense, providing healthy fats and vitamin E, widely used in African groundnut stews to combat protein-energy malnutrition. These examples illustrate how legumes are integrated into diverse cuisines, delivering essential nutrients and supporting health across socioeconomic contexts, from rural communities to urban vegan diets.
Conclusion
Legumes are undeniably nutritionally important, offering a robust combination of protein, fiber, complex carbohydrates, and micronutrients that address diverse dietary needs. Their health benefits, including cardiovascular protection, diabetes management, and anemia prevention, underscore their role in global nutrition. Examples like soybeans, lentils, chickpeas, and peanuts highlight their versatility and cultural significance, making them indispensable in both traditional and modern diets. As affordable, sustainable, and nutrient-dense foods, legumes continue to play a critical role in promoting health and combating malnutrition worldwide.
Question:-6(b)
Write a detailed note on economic importance of spices.
Answer:
Comprehensive Solution: Economic Importance of Spices
1. Role in Global Trade and Economy
Spices, derived from plant parts such as seeds, bark, roots, or fruits, are economically significant due to their pivotal role in global trade and commerce. Historically, spices like black pepper, cinnamon, and cloves drove the spice trade, connecting Asia, Africa, and Europe, and fueling economic prosperity in regions like India and Indonesia. Today, the global spice market is valued at over $20 billion annually, with India as the largest producer and exporter, contributing spices like turmeric, cumin, and cardamom. Spices generate substantial foreign exchange; for instance, India’s spice exports earned $4 billion in 2023, supporting millions of farmers and traders. Countries like Vietnam (pepper) and Sri Lanka (cinnamon) rely on spices as key economic drivers, boosting GDP and rural livelihoods. The demand for spices in food, cosmetics, and pharmaceutical industries ensures their continued economic relevance, with trade networks fostering international cooperation and economic stability.
2. Employment and Livelihood Support
Spices significantly contribute to employment, particularly in agrarian economies where cultivation, harvesting, and processing are labor-intensive. In India, the spice industry employs over 10 million people, including farmers, laborers, and workers in processing units. For example, saffron cultivation in Jammu and Kashmir provides livelihoods for thousands of families, with each kilogram of saffron requiring meticulous hand-harvesting of 150,000 crocus flowers. In Ethiopia, spice farming, including ginger and coriander, supports rural communities, reducing poverty and migration. Smallholder farmers in countries like Guatemala (cardamom) benefit from spice crops, which offer higher returns per hectare than staple crops like maize. The spice value chain, encompassing drying, grading, packaging, and marketing, creates diverse job opportunities, empowering women and youth in rural areas. This employment generation strengthens local economies and promotes sustainable development in spice-producing regions.
3. Applications in Industries
The economic importance of spices extends beyond agriculture to multiple industries, enhancing their commercial value. In the food industry, spices like chili, paprika, and cumin are essential for flavoring processed foods, snacks, and culinary products, driving a multi-billion-dollar market. For instance, the global demand for chili powder supports large-scale production in India and China. In pharmaceuticals, spices such as turmeric (containing curcumin) are used for their anti-inflammatory and antioxidant properties, fueling a growing nutraceutical sector valued at $400 billion globally. The cosmetic industry utilizes spices like saffron and clove in skincare and perfumery, with saffron-based creams commanding premium prices. Spices also have industrial applications, such as nutmeg and mace in essential oil production, used in aromatherapy and flavor extracts. These diverse applications create economic opportunities across manufacturing, research, and marketing, amplifying the economic footprint of spices.
4. Contribution to Cultural and Culinary Tourism
Spices contribute to economic growth through cultural and culinary tourism, attracting visitors to spice-producing regions. Spice tourism, such as tours of pepper plantations in Kerala, India, or vanilla farms in Madagascar, generates revenue for local economies. For example, Zanzibar’s “Spice Island” tours, showcasing cloves and nutmeg, draw thousands of tourists annually, supporting hospitality and artisanal sectors. Culinary festivals, like the Turmeric Festival in Telangana, India, promote local spices, boosting sales and cultural heritage. These activities enhance the economic value of spices by integrating them into tourism, creating income streams for farmers, guides, and small businesses while preserving traditional knowledge and practices.
Conclusion
Spices are economically vital, driving global trade, supporting livelihoods, and fueling diverse industries. Their role in earning foreign exchange, as seen in India’s spice exports, underscores their commercial significance. By providing employment to millions, particularly in rural areas, spices reduce poverty and empower communities. Their applications in food, pharmaceuticals, and cosmetics create robust markets, while spice tourism enhances cultural and economic value. As versatile and high-value commodities, spices continue to shape economies, blending tradition with modern commerce to sustain growth and development worldwide.
Question:-7(a)
Plant growth regulators form an important component of plant tissue culture medium. Justify the statement.
Answer:
Comprehensive Solution: Importance of Plant Growth Regulators in Plant Tissue Culture Medium
1. Role in Regulating Cell Division and Differentiation
Plant growth regulators (PGRs), also known as plant hormones, are critical components of plant tissue culture media due to their ability to control cellular processes essential for in vitro plant growth. PGRs, such as auxins, cytokinins, gibberellins, abscisic acid, and ethylene, regulate cell division, elongation, and differentiation in cultured explants. Auxins, like indole-3-acetic acid (IAA), promote cell division and root initiation, crucial for establishing callus or organogenesis. For example, in tobacco tissue culture, auxins induce dedifferentiation of explants into a callus, a mass of undifferentiated cells. Cytokinins, such as benzylaminopurine (BAP), stimulate shoot formation by promoting cell division in shoot meristems. The balance between auxins and cytokinins determines the developmental pathway; a high auxin-to-cytokinin ratio favors rooting, while a high cytokinin-to-auxin ratio promotes shoot formation, as seen in banana micropropagation. This precise control over cellular fate ensures successful regeneration of whole plants from explants, making PGRs indispensable in tissue culture.
2. Facilitation of Morphogenesis and Organogenesis
PGRs are vital for inducing morphogenesis, the process by which cultured tissues develop into organized structures like shoots, roots, or somatic embryos. In tissue culture, explants lack the natural hormonal signals of intact plants, necessitating exogenous PGRs to trigger organogenesis or embryogenesis. Gibberellins, for instance, enhance shoot elongation and are used in orchid cultures to promote plantlet growth. In somatic embryogenesis, auxins like 2,4-dichlorophenoxyacetic acid (2,4-D) induce embryogenic callus formation in crops like maize, while cytokinins support embryo maturation. Abscisic acid (ABA) plays a role in embryo development and stress tolerance, ensuring viable plantlets. For example, in coffee tissue culture, ABA is used to synchronize somatic embryo maturation. The specific combination and concentration of PGRs in the medium dictate the type of morphogenesis, enabling tailored protocols for different species. This ability to direct tissue development underscores PGRs’ importance in achieving desired outcomes in plant tissue culture.
3. Enhancement of Culture Efficiency and Viability
PGRs enhance the efficiency and viability of tissue cultures by optimizing growth conditions and overcoming physiological barriers. They prevent senescence and browning of explants, which can hinder culture success. For instance, cytokinins delay senescence in leafy explants, maintaining tissue viability during culture. PGRs also mitigate stress responses in vitro, such as hyperhydricity, by regulating water uptake and cell expansion. Ethylene inhibitors, like silver nitrate, are used in some cultures to prevent abnormal growth caused by ethylene accumulation. Additionally, PGRs improve the multiplication rate in micropropagation; in potato tissue culture, BAP increases shoot proliferation, enabling mass production of plantlets. By fine-tuning PGR concentrations, researchers can achieve high regeneration rates and reduce culture time, making protocols cost-effective. This optimization is critical for commercial applications, such as producing disease-free plants or conserving endangered species, highlighting PGRs’ role in culture success.
Conclusion
Plant growth regulators are indispensable in plant tissue culture, serving as master regulators of cell division, differentiation, and morphogenesis. Their ability to direct organogenesis and embryogenesis enables the regeneration of whole plants from explants, a cornerstone of tissue culture applications. By enhancing culture efficiency, preventing senescence, and optimizing growth, PGRs ensure viable and scalable protocols for micropropagation, genetic engineering, and conservation. Their precise manipulation in culture media underpins advancements in agriculture and biotechnology, making them a vital component of plant tissue culture systems.
Question:-7(b)
Discuss the role of plant tissue culture in germplasm conservation.
Answer:
Comprehensive Solution: Role of Plant Tissue Culture in Germplasm Conservation
1. Preservation of Genetic Diversity
Plant tissue culture plays a pivotal role in germplasm conservation by preserving the genetic diversity of plant species, particularly those that are endangered, rare, or economically important. Germplasm, encompassing seeds, tissues, or whole plants, represents the genetic material essential for breeding and biodiversity maintenance. Tissue culture techniques, such as in vitro storage and micropropagation, allow the conservation of plant genotypes that are difficult to preserve through traditional seed banking due to recalcitrant seeds (seeds that cannot be dried or frozen) or vegetative propagation limitations. For example, species like coconut and cacao have recalcitrant seeds, making tissue culture a vital tool for their conservation. By culturing explants like meristems or embryos in sterile conditions, tissue culture ensures the maintenance of genetic integrity without the risk of cross-pollination or genetic drift. This is critical for conserving wild relatives of crops, such as wild rice (Oryza rufipogon), which harbor valuable genes for disease resistance. In vitro conservation thus safeguards biodiversity, supporting future breeding and ecological restoration efforts.
2. Cryopreservation for Long-Term Storage
Cryopreservation, a specialized tissue culture technique, is instrumental in the long-term conservation of plant germplasm. This method involves storing plant tissues, such as shoot tips, embryos, or callus, at ultra-low temperatures (typically -196°C in liquid nitrogen) to halt metabolic activity while preserving genetic stability. Cryopreservation is particularly effective for species with limited seed viability or those propagated vegetatively, such as banana and potato. The process begins with treating explants with cryoprotectants, like dimethyl sulfoxide (DMSO), to prevent ice crystal formation during freezing. Techniques like vitrification, where tissues are dehydrated and rapidly frozen, enhance survival rates. For instance, the International Potato Center in Peru uses cryopreservation to conserve thousands of potato accessions, ensuring their availability for future generations. Cryopreservation minimizes the need for continuous in vitro subculturing, reducing the risk of somaclonal variation (genetic mutations during culture) and labor costs. This technique is a cornerstone of germplasm banks, securing plant genetic resources for decades or centuries.
3. Facilitation of Germplasm Exchange and Restoration
Plant tissue culture facilitates the safe exchange and restoration of germplasm, overcoming geographical and phytosanitary barriers. In vitro cultures, being sterile, allow the international transfer of plant material without the risk of introducing pests or pathogens, adhering to quarantine regulations. For example, disease-free banana plantlets produced via tissue culture are exported globally, supporting agriculture in tropical regions. Tissue culture also enables the mass propagation of conserved germplasm for reintroduction into natural habitats or agricultural systems. In conservation programs, tissue-cultured plantlets of endangered species, such as the pitcher plant (Nepenthes rajah), are reintroduced to restore populations in their native ecosystems. Additionally, tissue culture supports the multiplication of elite genotypes with desirable traits, such as drought-tolerant sorghum varieties, for distribution to farmers. This role in exchange and restoration enhances global access to genetic resources, promoting food security and biodiversity conservation.
Conclusion
Plant tissue culture is a cornerstone of germplasm conservation, offering innovative solutions to preserve plant genetic diversity. By enabling in vitro storage, it protects rare and recalcitrant species, while cryopreservation ensures long-term genetic stability. Its facilitation of safe germplasm exchange and restoration supports global agriculture and ecological restoration. As biodiversity faces threats from climate change and habitat loss, tissue culture remains an indispensable tool, safeguarding plant genetic resources for future breeding, conservation, and sustainable development.
Question:-8
Describe the technique of Agrobacterium-mediated transformation with the help of a diagram.
Answer:
Comprehensive Solution: Agrobacterium-Mediated Transformation Technique
1. Overview of Agrobacterium-Mediated Transformation
Agrobacterium-mediated transformation is a widely used biotechnological technique for introducing foreign genes into plant genomes, leveraging the natural genetic engineering ability of the soil bacterium Agrobacterium tumefaciens. This bacterium causes crown gall disease in plants by transferring a segment of its DNA, known as T-DNA (transfer DNA), into the host plant’s genome. Scientists exploit this mechanism to insert desired genes, such as those for pest resistance or herbicide tolerance, into plants. The technique is preferred for its efficiency, simplicity, and ability to produce stable, heritable transformations in a wide range of dicotyledonous and some monocotyledonous plants, including crops like tobacco, soybean, and rice. The process involves several steps, including bacterial preparation, gene construct design, plant tissue infection, selection of transformed cells, and plant regeneration, each requiring precise control to ensure successful transformation.
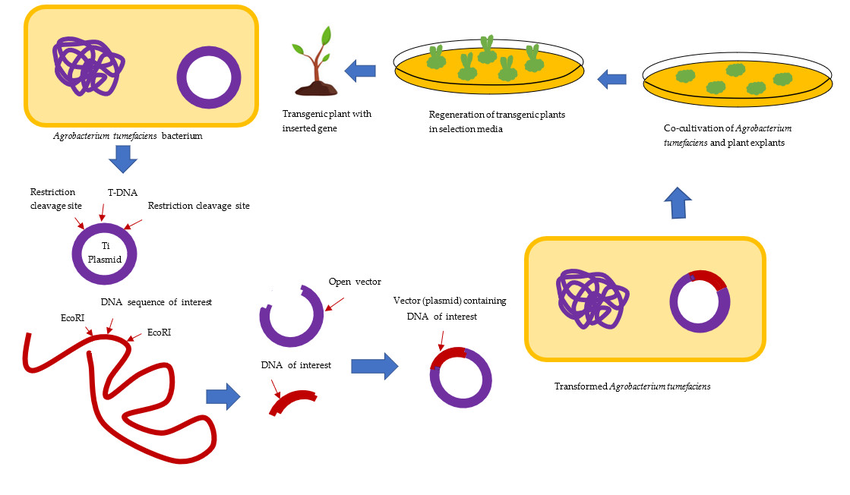
2. Steps in Agrobacterium-Mediated Transformation
Preparation of Agrobacterium and Gene Construct
The process begins with preparing Agrobacterium tumefaciens harboring a modified plasmid containing the T-DNA. The T-DNA is engineered to carry the gene of interest (e.g., a Bt toxin gene for insect resistance) flanked by left and right border sequences, which define the T-DNA region. The plasmid also includes a selectable marker gene (e.g., antibiotic or herbicide resistance) to identify transformed plant cells and virulence (vir) genes to facilitate T-DNA transfer. The native tumor-inducing genes are removed to prevent gall formation. The recombinant plasmid is introduced into Agrobacterium via electroporation, and the bacteria are cultured to achieve optimal density for infection.
Plant Tissue Preparation and Co-Cultivation
Plant tissues, typically explants like leaf discs, stems, or embryonic callus, are prepared for transformation. Common targets include tobacco leaf discs or rice callus due to their regenerative potential. The explants are surface-sterilized to eliminate contaminants and pre-cultured to enhance susceptibility to infection. The Agrobacterium suspension, activated with acetosyringone (a phenolic compound that induces vir genes), is co-cultivated with the explants. During co-cultivation, usually lasting 2–3 days at 25°C in the dark, Agrobacterium attaches to plant cells, and the T-DNA is transferred into the plant cell nucleus. A diagram of this step would depict Agrobacterium injecting T-DNA into a plant cell, with vir proteins facilitating the process and border sequences guiding integration.
T-DNA Transfer and Integration
The T-DNA transfer is mediated by vir proteins encoded by the Agrobacterium plasmid. Upon activation, vir genes produce proteins that process the T-DNA, forming a single-stranded T-strand coated with VirD2 and VirE2 proteins. This complex is transported into the plant cell via a type IV secretion system and enters the nucleus, where the T-DNA integrates randomly into the plant genome via non-homologous recombination. The selectable marker gene ensures that only transformed cells survive subsequent selection steps.
Selection and Regeneration of Transformed Plants
After co-cultivation, explants are washed to remove excess Agrobacterium and transferred to a selection medium containing antibiotics (e.g., kanamycin) or herbicides to eliminate non-transformed cells. The medium also includes antibiotics like cefotaxime to kill residual Agrobacterium. Transformed cells, expressing the marker gene, survive and form callus or shoots. These are cultured on regeneration media with plant growth regulators (e.g., auxins and cytokinins) to induce shoot and root formation, eventually producing whole transgenic plants. For example, in cotton transformation, transformed calli regenerate into plantlets over several weeks.
Verification of Transformation
Regenerated plants are tested to confirm T-DNA integration and gene expression using techniques like PCR, Southern blotting, or RT-PCR. Phenotypic assays, such as insect resistance tests for Bt-transformed plants, verify functionality. Transgenic plants are grown to maturity, and their progeny are analyzed to ensure stable inheritance of the introduced gene.
Conclusion
Agrobacterium-mediated transformation is a cornerstone of plant biotechnology, enabling precise genetic modifications for improved crop traits. By harnessing Agrobacterium’s natural ability to transfer T-DNA, the technique efficiently introduces genes into plant genomes, followed by selection and regeneration of transgenic plants. Its versatility and reliability make it indispensable for developing genetically modified crops like Bt maize or herbicide-tolerant soybean, advancing agriculture and food security.
Question:-9
Describe the morphology, cultivation and uses of any two fibre yielding plants.
Answer:
Comprehensive Solution: Morphology, Cultivation, and Uses of Two Fibre-Yielding Plants
1. Morphology of Cotton and Jute
Cotton (Gossypium hirsutum)
Cotton, a leading fibre-yielding plant, belongs to the Malvaceae family and is characterized by its shrubby, annual, or perennial growth, reaching 0.5–1.5 meters in height. The plant has broad, lobed leaves with 3–5 lobes, palmately veined, and a hairy surface. Its taproot system penetrates deep into the soil, aiding water uptake. The flowers are large, creamy-white or yellow, with five petals and a central reproductive column. The fruit, a capsule or boll, splits open at maturity to reveal seeds covered with soft, white fibres (lint) and shorter fuzz. These fibres, composed of cellulose, are the primary economic product, varying in length (20–40 mm) and fineness depending on the cultivar. Stems are erect, branched, and slightly woody, supporting the plant’s structure.
Jute (Corchorus olitorius and Corchorus capsularis)
Jute, from the Tiliaceae family, is an annual herbaceous plant growing 2–4 meters tall. It has a slender, straight stem with minimal branching, covered in fine hairs. The leaves are simple, alternate, lanceolate, with serrated margins and a glossy surface. Jute’s small, yellow flowers form clusters, producing elongated, ribbed capsules containing numerous seeds. The bast fibres, extracted from the stem’s phloem, are long (1–3 meters), lustrous, and yellowish-white, composed of cellulose and lignin. The root system is shallow but extensive, anchoring the plant in wet soils. Jute’s morphology, particularly its fibre-rich stem, makes it ideal for industrial applications.
2. Cultivation of Cotton and Jute
Cotton Cultivation
Cotton thrives in tropical and subtropical climates, requiring temperatures of 20–30°C, annual rainfall of 600–1,200 mm, and well-drained loamy soils with a pH of 6–7.5. Major producers include India, China, and the USA. Seeds are sown during spring (e.g., April–May in India) at a depth of 2–3 cm, with spacing of 60–90 cm between rows. Irrigation is critical in dry regions, supplemented by fertilizers rich in nitrogen and phosphorus. Pests like bollworms are managed with integrated pest management or Bt-cotton varieties. The crop matures in 150–180 days, with bolls harvested manually or mechanically when they split open. Defoliants may be applied to ease harvesting. Post-harvest, fibres are ginned to separate lint from seeds, ensuring quality for textile production.
Jute Cultivation
Jute is cultivated in hot, humid tropical regions, requiring temperatures of 24–35°C, high humidity, and annual rainfall of 1,000–2,000 mm. It grows best in alluvial, loamy soils with a pH of 5.5–7, as seen in the Ganges Delta of India and Bangladesh, the largest producers. Seeds are sown during the monsoon (March–April) by broadcasting or line sowing. The crop requires frequent irrigation and nitrogen-rich fertilizers to enhance fibre yield. Weeding is essential in early stages to reduce competition. Jute matures in 120–150 days, harvested when 50% of capsules form, by cutting stems at the base. Retting, a process of soaking stems in water for 10–20 days, separates fibres by microbial degradation of pectin, followed by stripping and drying.
3. Uses of Cotton and Jute
Cotton Uses
Cotton fibres are prized for their softness, durability, and breathability, making them the backbone of the global textile industry. Long-staple cotton, like Egyptian varieties, is used for high-quality fabrics, including clothing (shirts, jeans), bed linens, and towels. Short-staple fibres are used in lower-grade textiles or blended fabrics. Cottonseed oil, extracted from seeds, is used in cooking and food processing, while seed cake serves as animal feed. Cotton linters (short fibres) are used in paper, plastics, and explosives (nitrocellulose). The global cotton market, valued at $40 billion, supports economies in countries like India, employing millions in farming and textile sectors.
Jute Uses
Jute, known as the “golden fibre,” is valued for its strength and eco-friendliness. It is primarily used for manufacturing sacks, bags, and burlap for packaging agricultural goods like grains and coffee. Jute’s versatility extends to carpets, rugs, mats, and geotextiles for soil erosion control. In recent years, jute has gained traction in eco-friendly products like shopping bags and biodegradable composites, reducing plastic use. Jute sticks are used as fuel or in paper production. The jute industry, particularly in India and Bangladesh, generates significant revenue, with exports worth $1 billion annually, supporting rural livelihoods.
Conclusion
Cotton and jute, as fibre-yielding plants, are vital to global agriculture and industry. Cotton’s soft, versatile fibres dominate textiles, while jute’s strong, biodegradable fibres cater to packaging and eco-friendly products. Their distinct morphologies enable efficient fibre extraction, and their cultivation supports millions of livelihoods in tropical regions. The diverse uses of cotton and jute, from clothing to sustainable materials, underscore their economic and environmental significance, ensuring their continued relevance in modern economies and sustainable development.
Question:-10(a)
Differentiate between Northern and Western blotting.
Answer:
Differentiating Northern and Western Blotting
1. Purpose and Target Molecule
Northern and Western blotting are molecular biology techniques used to detect specific biomolecules, but they differ fundamentally in their target molecules and purposes. Northern blotting is designed to detect and analyze RNA molecules, particularly mRNA, to study gene expression levels or RNA processing. It is used to quantify specific RNA transcripts in a sample, for instance, to assess gene expression in plant tissues under stress. Western blotting, in contrast, targets proteins to study their expression, size, or post-translational modifications. It is widely used to confirm protein presence, such as detecting a stress-response protein in Arabidopsis. The distinction in molecular targets—RNA for Northern and proteins for Western—shapes their respective protocols and applications, making them complementary tools in molecular research.
2. Sample Preparation and Separation
The preparation and separation methods for Northern and Western blotting are tailored to their target molecules. In Northern blotting, total RNA is extracted from cells using methods like TRIzol, and mRNA may be enriched. The RNA sample is separated by size using agarose gel electrophoresis under denaturing conditions (e.g., with formaldehyde) to prevent secondary structures. Smaller RNA fragments migrate faster, creating a size-based profile. In Western blotting, proteins are extracted, often denatured with SDS, and separated by SDS-PAGE (polyacrylamide gel electrophoresis). Proteins are separated based on molecular weight, with smaller proteins migrating faster through the gel. These differences in sample handling and electrophoresis reflect the chemical properties of RNA (fragile, single-stranded) versus proteins (diverse, often folded).
3. Detection and Probing
Detection in Northern and Western blotting involves transferring separated molecules to a membrane and probing with specific molecules, but the probes and methods differ. In Northern blotting, RNA is transferred to a nylon membrane and hybridized with a labeled DNA or RNA probe complementary to the target RNA sequence. Probes are often radioactively or fluorescently labeled, and hybridization occurs under stringent conditions to ensure specificity. In Western blotting, proteins are transferred to a PVDF or nitrocellulose membrane and detected using antibodies. A primary antibody binds the target protein, followed by a secondary antibody conjugated to an enzyme (e.g., horseradish peroxidase) for signal amplification, visualized via chemiluminescence or colorimetric methods. These distinct probing strategies highlight the molecular specificity required for RNA versus protein detection.
Conclusion
Northern and Western blotting are specialized techniques for analyzing RNA and proteins, respectively, each critical for molecular biology research. Northern blotting excels in studying gene expression through RNA detection, while Western blotting provides insights into protein expression and modifications. Their differences in sample preparation, separation, and detection methods reflect the unique properties of their target molecules. Together, these techniques enable comprehensive analysis of gene and protein dynamics, advancing our understanding of biological processes in plants and other organisms.
Question:-10(b)
Write short note on rhizogenesis and caulogenesis.
Answer:
Short Note on Rhizogenesis and Caulogenesis
1. Rhizogenesis
Rhizogenesis refers to the process of root formation in plants, either naturally or induced in vitro during plant tissue culture. It is a critical step in plant propagation and regeneration, enabling plants to absorb water and nutrients. In tissue culture, rhizogenesis is induced by manipulating plant growth regulators, particularly auxins like indole-3-acetic acid (IAA) or indole-3-butyric acid (IBA), which promote root primordia development in explants such as stem cuttings or callus. For example, in micropropagation of banana, high auxin concentrations in the culture medium stimulate root initiation from shoot bases. The process involves cell dedifferentiation, followed by the formation of meristematic root initials that develop into adventitious or lateral roots. Rhizogenesis is essential for producing complete plantlets in vitro, ensuring their survival upon transfer to soil. It also supports clonal propagation and conservation of species with poor natural rooting, enhancing agricultural and horticultural applications.
2. Caulogenesis
Caulogenesis is the process of shoot formation or regeneration, occurring naturally or induced in tissue culture. It is vital for micropropagation, genetic transformation, and plant regeneration from explants like leaves, stems, or callus. In vitro, caulogenesis is triggered by cytokinins, such as benzylaminopurine (BAP), which promote cell division and shoot meristem formation. A high cytokinin-to-auxin ratio in the culture medium favors shoot initiation, as seen in tobacco leaf explant cultures where BAP induces multiple shoots. The process begins with the activation of meristematic cells, leading to the development of shoot primordia that elongate into shoots. Caulogenesis is crucial for mass propagation of elite plant varieties, such as orchids, and for regenerating transgenic plants after gene insertion. It enables the production of genetically uniform plantlets, supporting commercial horticulture and conservation efforts.
Conclusion
Rhizogenesis and caulogenesis are fundamental processes in plant tissue culture, driving root and shoot formation, respectively. Rhizogenesis, induced by auxins, ensures root development for nutrient uptake, while caulogenesis, driven by cytokinins, facilitates shoot regeneration for propagation. Both processes are critical for producing complete, viable plants in vitro, supporting applications in agriculture, biotechnology, and conservation by enabling mass propagation and genetic improvement of plants.